Effects of cement augmentation on the mechanical stability of multilevel spine after vertebral compression fracture
Introduction
Osteoporosis is the leading cause of non-traumatic vertebral compression fractures (1), and is responsible for approximately 750,000 vertebral fractures per annum in the United States alone (2). Conservative management involving bed rest, analgesia, external fixation and rehabilitation is effective for the majority of patients (3); however, about one-third will remain refractory, suffering from chronic pain (4), immobility, kyphotic deformity, decline in respiratory function and deterioration in quality of life (5).
Cement augmentation, or percutaneous vertebroplasty, is a minimally invasive treatment option that is reported to achieve pain relief (6-9) and stabilization of the fracture site (10). The procedure involves fluoroscopic- or CT-guided injection of acrylic cement, usually polymethylmethacrylate (PMMA) into the fractured vertebral body via a transpedicular or extrapedicular approach (11). As the incidence of vertebral compression fractures continues to rise as our population ages (12), cement augmentation will no doubt be performed more extensively, and more information is needed about this relatively new procedure.
Biomechanical studies of vertebroplasty have largely focused on the effects of cement augmentation on the strength and stiffness of single vertebrae, where the overall trend shows increased or maintained vertebral strength (13-26), and decreased or maintained stiffness (14-26) except when a large volume of cement was injected (13). In contrast, multi-level spine models that more closely approximate physiological conditions are limited and more heterogeneous. Across these studies, methods were less standardized, more varied parameters were examined, and results were less conclusive.
For instance, fracture generation has not been uniform: Hitchon et al. and Wilson et al. created wedge-compression fractures (27,28). Lu et al. created burst fractures (29), and Kanjaya et al. merely created a defect in the vertebral body to be filled by cement (30). Results also varied: Using 5-vertebrae spinal segments, Hitchon et al. found that range of motion (ROM) increased in all directions from the intact to the fractured state and was only restored in some directions after augmentation (27). Using 3-vertebrae segments, Lu et al. only examined flexion/extension (29) and reported increased stiffness post-augmentation. Wilson et al. only examined flexion/extension and lateral bending and reported reduced compliance (28) which also equates to increased stiffness as compliance is the reciprocal of stiffness (31). Contrastingly, Kanjaya et al. reported that stiffness and strength of 3-vertebrae segments were unaffected by augmentation and were dependent on mean vertebral bone mineral density (BMD) instead (30). Other multilevel studies explored adjacent vertebral failure attributable to vertebroplasty and did not examine kinematics of the spinal segment (32,33).
Further studies involving multi-vertebrae models are required to clarify the biomechanical effects of augmentation on spine kinematics. In this in vitro biomechanical study, we had 3 aims:
- To establish a 3-vertebrae ovine vertebral compression fracture model;
- To use the model to determine the effects of fracture on spine biomechanics;
- To discover whether cement augmentation can restore mechanical stability of the fractured spine.
Methods
Specimens
Five L1 to L3 lumbar spine segments were harvested from 5-year-old sheep, sealed and stored in a freezer at −20 °C. On the day of testing, the spines were thawed to room temperature and X-rayed using a high resolution X-ray (MX-20; Faxitron, Tucson, Arizona, USA) to exclude any major malformations. Excess soft tissue was excised, with care taken to ensure that no damage was done to the intervertebral discs or facet joint capsules. The interspinal ligaments, posterior longitudinal ligaments, and posterior elements of the vertebrae were retained, whereas portions of the transverse processes were excised to facilitate potting.
The cranial and caudal ends of each specimen were then embedded in aluminum boxes specific to the pure moment testing jig using casting resin (Smooth-Cast® 300 Series Bright White Liquid Plastic, Easton, Philadelphia, USA), with the L2 vertebral body and its 2 adjacent intervertebral discs left exposed, and positioned in such a way that the vertical axis of the spinal segment was vertically aligned with the loading axis. Retro-reflective markers were then attached to each corner of the metal boxes and one L2 transverse process (Figure 1).
Throughout the experiment, specimens were sprayed with phosphate buffer solution regularly.
Biomechanical testing
Each specimen was then mounted into a servo-hydraulic testing machine rig (MTS 858 Mini Bionix® machine or MTS 858 Bionix® machine, MTS System Inc., Eden Prairie, Minneapolis, USA), and tested using a validated pure moment testing system (34,35) (Figure 1). Pure moments of ±7.5 Nm were applied to each motion segment in axial rotation (AR), flexion/extension (FE) and lateral bending (LB) at ±0.25 Nm/s for 1 pre-conditioning cycle and 3 testing cycles in the testing machine with a 25kN axial-torsional load transducer (model number: 662.20D-05, MTS System Inc., Eden Prairie, Minneapolis, USA). Torque and angular motion were recorded at 20 Hz during testing. The last 3 cycles were used for data processing. In total, each specimen underwent the testing procedure 3 times: once intact, after fracture generation, and after cement augmentation.
A near infrared 3D motion tracking system (Cortex 4.0, Motion Analysis Corp., Santa Rosa, California, USA) employing the retro-reflective markers attached to the specimen was used to record motion of the spinal segment. Pretest calibration showed that the system was accurate to 0.1 mm in linear displacement and 0.1 in angular rotation. Post-processing was done with MATLAB (MathWorks, Natick, Massachusetts, USA) using an in-house written script to generate the torque-angle curves and corresponding range of motion (ROM) and neutral zone (NZ) values for the relative motions between levels L1 and L2 (L12), L1 and L3 (L13), and L2 and L3 (L23). ROM was defined as the deflection difference between the maximum applied loads in each direction (31), and NZ was defined as the angulation difference at zero load between the two directions of motion (36).
Fracture generation
To create uniform defects in all specimens, a handheld drill with a 3 mm drill bit was used to create a single row of 6 holes to weaken the cortical bone in the L2 vertebral body. The holes were placed 5 mm below the superior intervertebral disc, drilled to a depth of 5 mm, and each hole was spaced 2 mm apart (Figure 2). A plastic wedge with an angle of 10° was then secured to the superior surface of the potted specimen and the specimen was compressed using the same servo-hydraulic testing machine under displacement control at 30 mm/min to simulate a non-traumatic wedge-compression fracture (Figure 3). Compression was stopped once failure was achieved, and failure was defined as a drop in load with increasing displacement.
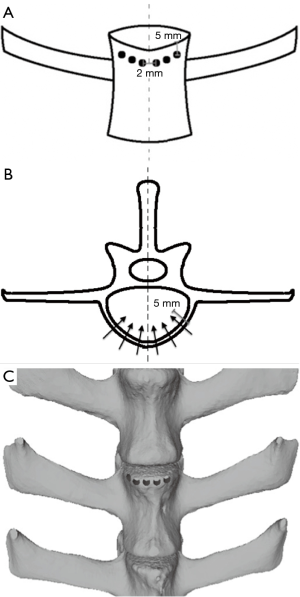
Cement augmentation
PMMA cement (Vertex Self-Curing, Vertex-Dental B.V., The Netherlands) mixed with 30% weight by weight barium sulphate powder, which conferred radiopacity to the cement without significant compromise in compressive strength, (37) was injected unipedicularly using an 11-gauge piercing needle connected to a 3-mL syringe into the vertebral centrum while the specimen was held in the pre-fracture neutral position. Each injection was started at 1 minute and 30 seconds after the monomer was added to the powder and was stopped when visible extrusion from the fracture site occurred. The cement was then allowed to set for at least an hour before undergoing testing.
Morphological analysis
Plain radiographs were taken when each specimen was intact, fractured and augmented. Fracture type was assessed according to the AOSpine thoracolumbar fractures classification system (38). Heights of the L1-2 intervertebral disc space were measured on lateral plain radiographs using the three-centerline method (39) with ImageJ computer software (National Institutes of Health, Bethesda, Maryland, USA) (Figure 4). To measure the disc space, 2 straight lines were drawn from 4 landmarks, A-D, chosen as the lowermost corners of the vertebral bodies adjacent to the disc space being measured (Figure 4). Three additional lines dividing the first two lines equally were drawn. Disc space was measured at sites 1, 2 and 3 using ImageJ (Figure 4), and the 3 lengths were averaged and scaled to obtain an approximation of the intervertebral disc space height.
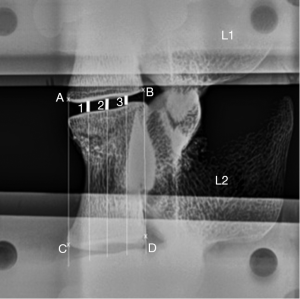
A transverse cut was made at the level of the augmentation after all testing has been completed to visualise cement distribution.
Statistical analysis
Using IBM SPSS Statistics Software (IBM, Armonk, New York, USA), one-way analysis of variance (ANOVA) was used to compare the data from the cyclic tests. The level of statistical significance was set at P<0.05 (CI: 95%).
Results
Biomechanical testing
Apart from a statistically significant increase in ROM of the fractured spine compared to the intact spine in AR between L12 (P=0.024), no significant difference in ROM or NZ was found between any of the other groups. The mean ROM and NZ values are listed in Tables 1,2 respectively.
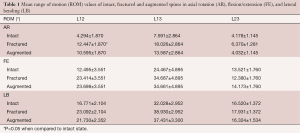
Full table
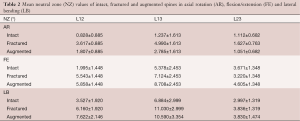
Full table
Despite the lack of statistically significant changes, there was still an appreciable trend showing increases in both ROM and NZ after fracture in all directions of motion. There was minimal return to normal biomechanics after augmentation. For ROM, augmentation resulted in small reductions in AR and LB, and almost no change for FE; whereas for NZ, augmentation resulted in a reduction in AR, and small increases in FE and LB. These results are represented in Figure 5, where the ROM and NZ for L12, which had similar trends to those of L13, were graphed. L23 did not share the same trends as this level was largely unaffected by the fracture and augmentation processes, as expected.
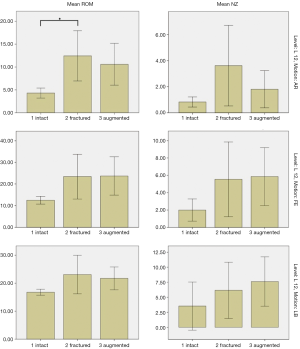
Morphologic analysis
Radiography revealed an anterior wedge-compression fracture involving the superior endplate only in the L2 vertebral body of all specimens (Figure 6). This type of fracture is classified as an A1 wedge-compression fracture according to the AO Spine Injury Classification System (38) and is the most common type of vertebral fractures, especially in osteoporotic elderly people (12,40). All gaps in the vertebral body were filled with cement with augmentation. No appreciable trend was observed for change in intervertebral disc height between the 3 states (Table 3). However, an increase in disc height after fracture, as evidenced in samples 1, 2 and 4, was associated with failure of bone adjacent to the disc space (Figure 7).
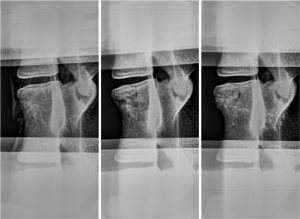
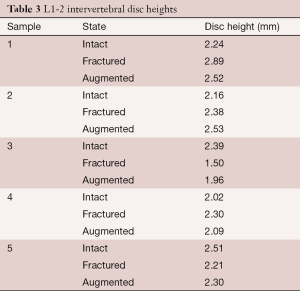
Full table
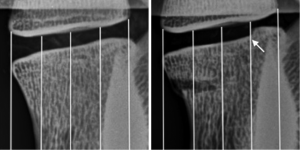
Transverse cut sections at the level of augmentation (Figure 8) revealed good cement dispersion into the fracture site; however, there was limited penetration into the cancellous bone.
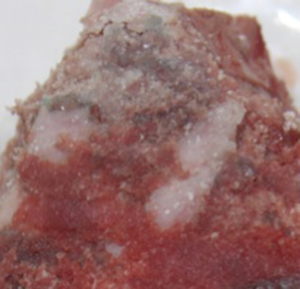
Discussion
This was the first study to use sheep spine to establish a model to explore the effects of vertebral compression fracture and cement augmentation on spine biomechanics. The experimental results show that both ROM and NZ increased in all directions of motion after fracture, and remained increased after augmentation. These trends were not statistically significant. Only the increase in ROM in AR after fracture between L12 was found to be of statistical significance (P=0.024) when compared to the intact state.
As mentioned in the introduction, similar studies have reported the effects of augmentation on multilevel spine by examining ROM (27), stiffness (29,30), compliance (28) and strength (8) The only previous study that examined ROM is Hitchon et al. using 5-vertebrae human spine (T9-L3) with the creation of a T11 compression fracture achieved via partial coring of the vertebral body and the weight-drop technique (27). While we did not differentiate between right and left AR, flexion, extension, and right and left LB, Hitchon et al. performed isolated analyses of these 6 modes of angular motion. Both studies report increased ROM in all directions of motion after fracture, but these increases reached statistical significance in Hitchon et al.’s study (27). That same study also reported that augmentation with both hydroxyapatite and PMMA reduced ROM in all directions (27), a finding which was not repeated in our study. In addition, Hitchon et al. included fatigue testing which was associated with increased rotation in all directions except extension (27).
To date, no other study has examined the effects of fracture and augmentation on NZ. In comparison to ROM, NZ has been considered a more direct estimation of spinal instability (41) and more sensitive in quantifying spinal destabilization such as that caused by sponylolisthesis (41,42), which we extended to quantifying destabilization caused by fracture. Despite these ostensible advantages, NZ is more difficult to interpret when multiple sites exhibit motion, and quantifying NZ is problematic (31) as several methods, each of which gives different values, can be employed to achieve this (31,35,36,43-45). This study utilized Wilke et al.’s method (36).
When interpreting this study, several considerations should be borne in mind. BMD measurements were not taken, and thus could not be factored in during fracture generation or statistical analysis. Also, all sheep used in this study were assumed to be healthy with normal BMD, whereas non-traumatic compression fractures in humans usually occur on pathological spine, most commonly in osteoporotic or metastatic vertebrae (1). Additionally, the augmentation procedure was not ideal. There was no fluoroscopic guidance, the cement injection was not pressurized and dental cement was used instead of bone cement. The use of unipedicular injection technique has also been found to produce significantly weaker vertebral bodies than bipedicular injections (23). Cement was also prone to anterior leakage out of the fracture site. As such, the volume injected was not recorded, but it was noted to be considerably less than the average 7 mL used in humans (46), which was expected as sheep have smaller vertebral bodies (47). Moreover, augmentation only achieved fracture repair; possible disc injury and other soft tissue injury were not treated or assessed, apart from measurements taken of the intervertebral disc height. Furthermore, the biomechanical test protocol may not have been ideal. Currently, the established load for testing sheep vertebrae is ±7.5 Nm (48,49), but this load was applied to intact spine, as opposed to fractured spine and may have been too large as 2 fractured specimens in the pilot study broke during testing. The vertebral body also split open at the fracture site in some specimens when moments were applied in extension and lateral bending, leading to possible overestimations of ROM and NZ in FE and LB.
Limitations of this study include the small sample size and the fact that an animal model was used. Biomechanical differences exist between human and sheep vertebrae, and BMD of sheep vertebra is about twice as high as in humans (50), however animal spines have less variation, and ROM of sheep spine in all load directions are qualitatively similar to human spine (49), hence sheep spines are well-accepted as suitable models for pre-clinical in vitro biomechanical tests (48). Another limitation was the high standard deviation in ROM and NZ of the fractured specimens despite efforts to generate a standardized wedge-compression fracture. Other limitations include the augmentation procedure and testing protocol as mentioned above. The spine used in this study was also from healthy sheep, as opposed to those with osteoporosis, which more closely mimic the human condition predisposing to vertebral compression fractures.
Future multivertebrae studies can consider incorporating follower load, an internal compressive load that markedly increases the load-carrying capacity of the in vitro spine and simulates the stabilizing role of axial muscles in vivo. (51). Follower load can be applied using a series of cables and dead weights to minimize internal shear forces and bending moments on the spine so that the resultant internal force becomes compressive and the load path approximates the tangent to the lumbar spine curvature (51). Follower load may also prevent splitting of the fractured vertebral body during cyclic testing. Future studies may also consider exploring the behavior of spinal fractures divided according to type, severity and/or mechanism, and effects of cement augmentation on each group.
Although cement augmentation was unable to restore normal biomechanics to the fractured spine in our study, model-specific factors, such as challenges faced during augmentation and cyclic testing, may have contributed this. Soft tissue damage was also identified as a likely contributor. Caution should therefore be used when applying these results to clinical scenarios. Regardless, ovine vertebrae are well accepted as models for pre-clinical biomechanical tests (48), and can be extended to study vertebral compression fractures that were successfully generated when well controlled for. In conclusion, this model should still be regarded as useful for evaluating fractured spine biomechanics.
Acknowledgements
We sincerely thank our colleagues from the Surgical and Orthopaedic Research Laboratories at Prince of Wales Hospital.
Footnote
Conflicts of Interest: The authors have no conflicts of interest to declare.
References
- Montagu A, Speirs A, Baldock J, et al. A review of vertebroplasty for osteoporotic and malignant vertebral compression fractures. Age Ageing 2012;41:450-5. [Crossref] [PubMed]
- Riggs BL, Melton LJ 3rd. The worldwide problem of osteoporosis: insights afforded by epidemiology. Bone 1995;17:505S-511S. [Crossref] [PubMed]
- Rapado A. General management of vertebral fractures. Bone 1996;18:191S-196S. [Crossref] [PubMed]
- Kim DH, Vaccaro AR. Osteoporotic compression fractures of the spine; current options and considerations for treatment. Spine J 2006;6:479-87. [Crossref] [PubMed]
- Hulme PA, Krebs J, Ferguson SJ, et al. Vertebroplasty and kyphoplasty: a systematic review of 69 clinical studies. Spine (Phila Pa 1976) 2006;31:1983-2001. [Crossref] [PubMed]
- Blasco J, Martinez-Ferrer A, Macho J, et al. Effect of vertebroplasty on pain relief, quality of life, and the incidence of new vertebral fractures: a 12-month randomized follow-up, controlled trial. J Bone Miner Res 2012;27:1159-66. [Crossref] [PubMed]
- Farrokhi MR, Alibai E, Maghami Z. Randomized controlled trial of percutaneous vertebroplasty versus optimal medical management for the relief of pain and disability in acute osteoporotic vertebral compression fractures. J Neurosurg Spine 2011;14:561-9. [PubMed]
- Kallmes DF, Comstock BA, Heagerty PJ, et al. A randomized trial of vertebroplasty for osteoporotic spinal fractures. N Engl J Med 2009;361:569-79. [Crossref] [PubMed]
- Rousing R, Hansen KL, Andersen MO, et al. Twelve-months follow-up in forty-nine patients with acute/semiacute osteoporotic vertebral fractures treated conservatively or with percutaneous vertebroplasty: a clinical randomized study. Spine (Phila Pa 1976) 2010;35:478-82. [Crossref] [PubMed]
- Levine SA, Perin LA, Hayes D, et al. An evidence-based evaluation of percutaneous vertebroplasty. Manag Care 2000;9:56-60, 63. [PubMed]
- Groen RJ, du Toit DF, Phillips FM, et al. Anatomical and pathological considerations in percutaneous vertebroplasty and kyphoplasty: a reappraisal of the vertebral venous system. Spine (Phila Pa 1976) 2004;29:1465-71. [Crossref] [PubMed]
- Ploeg WT, Veldhuizen AG. Percutaneous vertebroplasty as a treatment for osteoporotic vertebral compression fractures: a systematic review. Eur Spine J 2006;15:1749-58. [Crossref] [PubMed]
- Bai B, Jazrawi LM, Kummer FJ, et al. The use of an injectable, biodegradable calcium phosphate bone substitute for the prophylactic augmentation of osteoporotic vertebrae and the management of vertebral compression fractures. Spine (Phila Pa 1976) 1999;24:1521-6. [Crossref] [PubMed]
- Belkoff SM, Maroney M, Fenton DC, et al. An in vitro biomechanical evaluation of bone cements used in percutaneous vertebroplasty. Bone 1999;25:23S-26S. [Crossref] [PubMed]
- Belkoff SM, Mathis JM, Erbe EM, et al. Biomechanical evaluation of a new bone cement for use in vertebroplasty. Spine (Phila Pa 1976) 2000;25:1061-4. [Crossref] [PubMed]
- Belkoff SM, Mathis JM, Fenton DC, et al. An ex vivo biomechanical evaluation of an inflatable bone tamp used in the treatment of compression fracture. Spine (Phila Pa 1976) 2001;26:151-6. [Crossref] [PubMed]
- Belkoff SM, Mathis JM, Jasper LE. Ex vivo biomechanical comparison of hydroxyapatite and polymethylmethacrylate cements for use with vertebroplasty. AJNR Am J Neuroradiol 2002;23:1647-51. [PubMed]
- Belkoff SM, Mathis JM, Jasper LE, et al. An ex vivo biomechanical evaluation of a hydroxyapatite cement for use with vertebroplasty. Spine (Phila Pa 1976) 2001;26:1542-6. [Crossref] [PubMed]
- Belkoff SM, Mathis JM, Jasper LE, et al. The biomechanics of vertebroplasty. The effect of cement volume on mechanical behavior. Spine (Phila Pa 1976) 2001;26:1537-41. [Crossref] [PubMed]
- Galovich LA, Perez-Higueras A, Altonaga JR, et al. Biomechanical, histological and histomorphometric analyses of calcium phosphate cement compared to PMMA for vertebral augmentation in a validated animal model. Eur Spine J 2011;20 Suppl 3:376-82. [Crossref] [PubMed]
- Heini PF, Berlemann U, Kaufmann M, et al. Augmentation of mechanical properties in osteoporotic vertebral bones--a biomechanical investigation of vertebroplasty efficacy with different bone cements. Eur Spine J 2001;10:164-71. [Crossref] [PubMed]
- Kolb JP, Kueny RA, Püschel K, et al. Does the cement stiffness affect fatigue fracture strength of vertebrae after cement augmentation in osteoporotic patients? Eur Spine J 2013;22:1650-6. [Crossref] [PubMed]
- Tohmeh AG, Mathis JM, Fenton DC, et al. Biomechanical efficacy of unipedicular versus bipedicular vertebroplasty for the management of osteoporotic compression fractures. Spine (Phila Pa 1976) 1999;24:1772-6. [Crossref] [PubMed]
- Tomita S, Kin A, Yazu M, et al. Biomechanical evaluation of kyphoplasty and vertebroplasty with calcium phosphate cement in a simulated osteoporotic compression fracture. J Orthop Sci 2003;8:192-7. [Crossref] [PubMed]
- Turner TM, Urban RM, Singh K, et al. Vertebroplasty comparing injectable calcium phosphate cement compared with polymethylmethacrylate in a unique canine vertebral body large defect model. Spine J 2008;8:482-7. [Crossref] [PubMed]
- Zhu X, Chen X, Chen C, et al. Evaluation of calcium phosphate and calcium sulfate as injectable bone cements in sheep vertebrae. J Spinal Disord Tech 2012;25:333-7. [Crossref] [PubMed]
- Hitchon PW, Goel V, Drake J, et al. Comparison of the biomechanics of hydroxyapatite and polymethylmethacrylate vertebroplasty in a cadaveric spinal compression fracture model. J Neurosurg 2001;95:215-20. [PubMed]
- Wilson DR, Myers ER, Mathis JM, et al. Effect of augmentation on the mechanics of vertebral wedge fractures. Spine (Phila Pa 1976) 2000;25:158-65. [Crossref] [PubMed]
- Lu WW, Cheung KM, Li YW, et al. Bioactive bone cement as a principal fixture for spinal burst fracture: an in vitro biomechanical and morphologic study. Spine (Phila Pa 1976) 2001;26:2684-90; discussion 2690-1. [Crossref] [PubMed]
- Kayanja MM, Schlenk R, Togawa D, et al. The biomechanics of 1, 2, and 3 levels of vertebral augmentation with polymethylmethacrylate in multilevel spinal segments. Spine (Phila Pa 1976) 2006;31:769-74. [Crossref] [PubMed]
- Smit TH, van Tunen MS, van der Veen AJ, et al. Quantifying intervertebral disc mechanics: a new definition of the neutral zone. BMC Musculoskelet Disord 2011;12:38. [Crossref] [PubMed]
- Berlemann U, Ferguson SJ, Nolte LP, et al. Adjacent vertebral failure after vertebroplasty. A biomechanical investigation. J Bone Joint Surg Br 2002;84:748-52. [Crossref] [PubMed]
- Boger A, Heini P, Windolf M, et al. Adjacent vertebral failure after vertebroplasty: a biomechanical study of low-modulus PMMA cement. Eur Spine J 2007;16:2118-25. [Crossref] [PubMed]
- Pelletier MH, Cordaro N, Punjabi VM, et al. PEEK Versus Ti Interbody Fusion Devices: Resultant Fusion, Bone Apposition, Initial and 26-Week Biomechanics. Clin Spine Surg 2016;29:E208-14. [PubMed]
- Spenciner D, Greene D, Paiva J, et al. The multidirectional bending properties of the human lumbar intervertebral disc. Spine J 2006;6:248-57. [Crossref] [PubMed]
- Wilke HJ, Wenger K, Claes L. Testing criteria for spinal implants: recommendations for the standardization of in vitro stability testing of spinal implants. Eur Spine J 1998;7:148-54. [Crossref] [PubMed]
- Jasper LE, Deramond H, Mathis JM, et al. Material properties of various cements for use with vertebroplasty. J Mater Sci Mater Med 2002;13:1-5. [Crossref] [PubMed]
- Vaccaro AR, Oner C, Kepler CK, et al. AOSpine thoracolumbar spine injury classification system: fracture description, neurological status, and key modifiers. Spine (Phila Pa 1976) 2013;38:2028-37. [Crossref] [PubMed]
- Kim KS, Yoon ST, Li J, et al. Disc degeneration in the rabbit: a biochemical and radiological comparison between four disc injury models. Spine (Phila Pa 1976) 2005;30:33-7. [Crossref] [PubMed]
- Eastell R, Cedel SL, Wahner HW, et al. Classification of vertebral fractures. J Bone Miner Res 1991;6:207-15. [Crossref] [PubMed]
- Hasegewa K, Kitahara K, Hara T, et al. Biomechanical evaluation of segmental instability in degenerative lumbar spondylolisthesis. Eur Spine J 2009;18:465-70. [Crossref] [PubMed]
- Panjabi MM. Clinical spinal instability and low back pain. J Electromyogr Kinesiol 2003;13:371-9. [Crossref] [PubMed]
- Panjabi MM. The stabilizing system of the spine. Part II. Neutral zone and instability hypothesis. J Spinal Disord 1992;5:390-6; discussion 397. [Crossref] [PubMed]
- Sarver JJ, Elliott DM. Mechanical differences between lumbar and tail discs in the mouse. J Orthop Res 2005;23:150-5. [Crossref] [PubMed]
- Thompson RE, Barker TM, Pearcy MJ. Defining the Neutral Zone of sheep intervertebral joints during dynamic motions: an in vitro study. Clin Biomech (Bristol, Avon) 2003;18:89-98. [Crossref] [PubMed]
- Sagi H YH. Vertebroplasty and Kyphoplasty in the Treatment of Osteoporotic Vertebral Fractures. In: Haher TR MA, editor. Surgical Techniques for the Spine. New York, NY 10001: Thieme New York, 2003.
- Sheng SR, Wang XY, Xu HZ, et al. Anatomy of large animal spines and its comparison to the human spine: a systematic review. Eur Spine J 2010;19:46-56. [Crossref] [PubMed]
- Kettler A, Liakos L, Haegele B, et al. Are the spines of calf, pig and sheep suitable models for pre-clinical implant tests? Eur Spine J 2007;16:2186-92. [Crossref] [PubMed]
- Wilke HJ, Kettler A, Claes LE. Are sheep spines a valid biomechanical model for human spines? Spine (Phila Pa 1976) 1997;22:2365-74. [Crossref] [PubMed]
- Smit TH. The use of a quadruped as an in vivo model for the study of the spine - biomechanical considerations. Eur Spine J 2002;11:137-44. [Crossref] [PubMed]
- Patwardhan AG, Havey RM, Meade KP, et al. A follower load increases the load-carrying capacity of the lumbar spine in compression. Spine (Phila Pa 1976) 1999;24:1003-9. [Crossref] [PubMed]
Contributions: (I) Conception and design: All authors; (II) Administrative support: WR Walsh; (III) Provision of study materials or patients: WR Walsh; (IV) Collection and assembly of data: E Tan, T Wang; (V) Data analysis and interpretation: E Tan, T Wang, M Pelletier; (VI) Manuscript writing: All authors; (VII) Final approval of manuscript: All authors.