A single center retrospective clinical evaluation of anterior cervical discectomy and fusion comparing allograft spacers to silicon nitride cages
Introduction
Anterior cervical discectomy and fusion (ACDF) is a common corrective procedure for various degenerative spinal disorders including stenosis, disc herniation, spondylosis, osteophyte formation, and spondylolisthesis (1,2). Autologous bone harvested from the iliac crest has been traditionally utilized as a replacement spacer within the operative cervical segment (3). While the use of autograft is considered to be the “gold standard” in ACDF (1,4), it has also been associated with a long-history of chronic harvest-site maladies including pain, seromas, hematomas, infections, and fractures (5). Persistent patient complaints about these comorbidities have led practitioners to substitute allograft spacers or abiotic biomaterial cages (e.g., Ti-alloys, PEEK) for autograft (6). Indeed, there has been a remarkable shift from the use of autogenous and allograft spacers to abiotic cages over the past 20 years. In 1998, 86% of all ACDF cases used autograft. Allograft comprised just 14% of total implantations, whereas cage use was still in its infancy. By 2008, autograft had declined to 10%, while allograft and interbody cages increased to 59% and 31%, respectively (7). Today, interbody cages dominate with ~60% of the devices produced from PEEK and 10% from Ti-alloys, with the balance being primarily a mixture of autograft and allograft (8).
While there have been numerous clinical studies comparing the safety and efficacy of autograft, allograft, and abiotic cages through the years, there is still a paucity of strong evidence supporting any one material. Most studies collectively suggest the equivalence of autograft and allograft, but generally favor autograft because of earlier arthrodesis (9-12). Clinical evaluations or reviews have compared autograft to Ti-cages (13-16), autograft to PEEK-cages (17), allograft to Ti-cages (18), allograft to PEEK-cages (19), Ti- to PEEK-cages (20-22), or multiple interbody materials (1,23,24) and have found little or no differences in reported outcomes, fusion rates, or subsidence. While these studies generally conclude that iliac crest grafts are still the “gold standard” due to earlier and more robust fusions, they concede that abiotic cages containing autologous bone are acceptable lower-morbidity alternatives. This conclusion is not particularly surprising given the greater osteogenic character of autograft compared with denatured allograft or the abiological cages. In fact, the initial requirements for allograft and the abiotic cages excluded biological activity. They were accepted solely based on sterility, biocompatibility, and mechanical properties (25-27). It has only been during the past 5 years that alterations of these devices (e.g., composites and coatings) have been engineered to upregulate their osteogenic capabilities (28,29).
Silicon nitride (Si3N4) is a relatively new spinal arthrodesis cage that has favorable osteopromotive and bacteriostatic properties. It was selected for this comparative study because its characteristics appear ideal for an interbody device including biocompatibility (30-36), enhanced strength and fracture toughness (37,38), moderate radiolucency (39,40), osteoconductivity (41-48), and bacterial resistance (41,47,49-51). Si3N4 was first successfully used as an intervertebral device in a small clinical trial in Australia in 1986 (52), and a number of recent case and clinical studies have reported its favorable interbody characteristics (53-57).
The purpose of this study was to retrospectively assess ACDF outcomes for 58 patients (108 segments) who received a Si3N4 cage compared to 34 patients (61 segments) who were implanted with fibular allograft spacers all by the same surgeon (MWS). Fusion was assessed using lateral radiographs (normal, flexion, and extension) at 3, 6, 12, and 24 months post-operatively using ISD and Cobb angle methods. Assessments were also made for the extent of osseous integration, the presence of bridging bone, an absence of peri-implant radiolucencies, and subsidence. The null hypotheses assumed that there would be no significant differences for any of these outcomes.
Methods
This study was designed as a retrospective chart review of patients on whom a single fellowship-trained spine surgeon (MWS) performed ACDF with either a Si3N4 cage (Valeo™C-II, Amedica Corporation, Salt Lake City, UT) or a structural fibular allograft spacer between August 1, 2013 and December 31, 2016. Inclusion and exclusion criteria are listed in Table 1. The medical procedures reported herein were consistent with the standard-of-care for these types of cases and did not involve more than minimal risk to the patients. Institutional review board (IRB) approval was obtained; but due to the retrospective nature of the study, informed consent by the IRB was not required. Nevertheless, all patient information and data remain completely anonymous and in compliance with IRB standards.
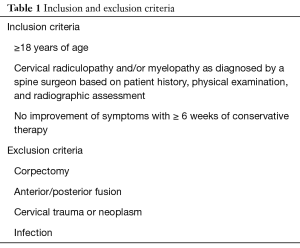
Full table
Surgical procedure
After radiographically localizing the affected level, a standard Smith-Robinson approach was made on the left-side (58). Complete discectomy, release of the posterior longitudinal ligament (PLL), and decompression of the uncinate process were then performed. A high-speed burr was used to prepare the endplates in a box-like fashion without violating their structural integrity. For all surgeries, burr shavings were harvested from local bone. The resulting autograft and DBM putty were pressed into either the endplates of a fibular allograft spacer or the center of a cage. After sizing, the bone spacers and Si3N4 cages, all lordotic, were placed at the appropriate levels. A standard anterior plate and screw construct was introduced for stabilization. Post-operatively, patients were mobilized as soon as possible. No cervical orthoses were used following surgery. The patients were instructed to restrict lifting to less than ~4.5 kg (<10 lbs.) during the first 6 weeks and no more than ~11.3 kg (25 lbs.) between weeks 6 and 12, as well as to avoid repetitive bending or twisting of the neck for at least 3 months.
Radiological assessment
Lateral radiographs were acquired at the following nominal post-operative intervals (within a margin of error): 2 weeks (<4 weeks); 6 weeks (≥4 and <8 weeks); 3 months (≥8 and <18 weeks); 6 months (≥18 and <40 weeks); 12 months (≥40 and <78 weeks); and 24 months (≥78 weeks) to assess fusion via visible osseous integration—defined as the presence of bridging bone and the absence of peri-implant radiolucency (55). These lateral radiographs were also used to evaluate subsidence—defined as the difference in segmental height (i.e., fused vertebrae plus disc space) between 2-week postoperative and subsequent follow-up radiographs (59). Additionally, flexion and extension radiographs at 3, 6, 12, and 24 months were used to assess fusion via ISD and Cobb angle methods (60,61). In the former method, nonunion at a given time-point was defined as a difference in ISD (i.e., between the spinous process tips of adjacent, fused vertebrae) of >2 mm between the patient’s flexion and extension radiographs, indicating excessive sagittal motion. Nonunion was similarly defined in the latter method as a difference in Cobb angle (i.e., between the line perpendicular to the top surface of the upper fused vertebral body and the line perpendicular to the bottom surface of the lower fused vertebral body) of >2° between the patient’s flexion and extension radiographs. Examples of these methods, applied to a Si3N4 cage, are provided in Figure 1. All radiographs were subsequently evaluated for fusion by the senior author and independently checked by the second author.
Outcome measures
The time to fusion using the ISD and Cobb methods was selected as the primary outcome measure. Secondary measures included fusion rate, osseous integration, and subsidence.
Statistical analyses
The Wilcoxon rank-sum test for ordinal data and Fisher’s exact test for categorical data were used for statistical analyses with α=0.05. All statistical analyses were run using commercially available statistical software (Minitab 18, Minitab Inc., State College, PA, USA).
Results
Between August of 2013 and December of 2016, fifty-eight patients underwent ACDF with Si3N4 cages (108 total levels) and thirty-four patients (61 total levels) underwent ACDF with allograft spacers. A patient accountability flowchart is given in Figure 2.
Demographics and patient characteristics
Demographics, comorbidities, and operative details are presented in Table 2. It should be noted that not all patients appeared for all of the follow-up periods; therefore, each evaluation time-point represents a slightly different patient population (cf., Figure 2). Data on a total of 52 patients and 101 operative levels were available at ≥12-month follow-up (i.e., 31 Si3N4 patients, 63 levels; and 21 allograft patients, 38 levels). There were no significant differences in patient demographics, comorbidities, or operative details between the two cohorts with the possible exception of a higher proportion of females in the Si3N4 group at ≥12-month follow-up (cf., Table 2). The majority of patients in both cohorts were overweight or obese with a primary diagnosis of radiculopathy or a combination of radiculopathy and myelopathy. About 50% were smokers and a significant percentage was also diabetic (i.e., between ~22% and 41%).
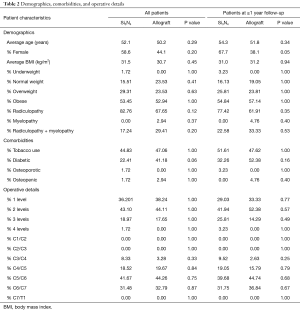
Full table
Complications and reoperations
Complication rates for the two cohorts are provided in Table 3. The surgical procedure and hospital stay were uneventful for most of the patients. Seven patients from the Si3N4 group and four patients from the allograft group suffered from recurrent dysphagia or dysphonia for more than six weeks. All were resolved within three months of follow-up using Medrol dospaks (Pfizer, NY, USA). Five Si3N4 patients and one allograft patient underwent subsequent ACDF operations for adjacent level disorders (i.e., stenosis with radiculopathy) that were not symptomatic at the time of their index procedures. Additionally, a recurrence of symptoms (e.g., persistent pain or paresthesia) was the sole reason for three revision surgeries in the Si3N4 cohort, whereas nonunion (five patients) and a post-operative infection (one patient) were reasons for revisions in the allograft cohort. In the latter case, Staphylococcus aureus was identified as the causative pathogen. All revisions resulted in solid arthrodesis and resolution of pain.
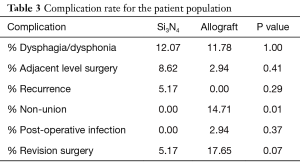
Full table
Clinical outcomes
Primary and secondary outcomes are presented in Table 4 and in Figures 3-6. For the primary measure of time to fusion, both the ISD and Cobb angle methods demonstrated that the Si3N4 cages provided earlier arthrodesis, averaging ~4 and ~7 months, respectively. This contrasted with the fusion assessments for the allograft group of ~9 and ~12 months, respectively. The difference in time to fusion between the two cohorts for both measurement methods was statistically significant (P=0.01 and 0.04, cf., Table 4). All of the secondary outcomes also favored Si3N4 over allograft. At ≥12-month follow-up, ~97% and ~87% of levels were fused in the Si3N4 and allograft groups using the ISD method, respectively (P=0.10, cf., Table 4). This compares to ~84% and ~68% using the Cobb angle method, respectively (P=0.07, cf., Table 4). The percent of osseous integration for the Si3N4 group was ~94% compared to ~76% for allograft (P=0.02, cf., Table 4) and the time for osseous integration was ~8 months for the Si3N4 cohort compared to ~19 months for the allograft group (P=0.00, cf., Table 4). Average subsidence after at least 1-year follow-up was 0.51 and 2.71 mm for the Si3N4 cages and allograft spacers, respectively, (P=0.00, cf., Table 4). The time-series results at the earlier follow-up periods are shown in Figures 3 through 6 for fusion rate using ISD and Cobb angle methods, osseous integration, and subsidence, respectively. At each time-point, these data showed that the Si3N4 cohort had earlier and more effective fusion (cf., Figures 3,4), greater osseous integration (cf., Figure 5), and lower subsidence (cf., Figure 6). With the exception of the Cobb angle measurements for fusion rate, all of the data for fusion rate and % osseous integration at 3, 6, and 12 months were significant. The subsidence results also favored the Si3N4 group at every follow-up time-point (cf., Figure 6). At 24-month follow-up, 100% of the patients in the Si3N4 group were fused compared to ~96% of the allograft group using the ISD method (P=1.00, cf., Figure 3), whereas for the Cobb method, ~95% and ~81% fusion results were observed, respectively (P=0.35, cf., Figure 4). A representative radiographic example of the progressive fusion of a single level ACDF using a Si3N4 cage is shown in Figure 7.
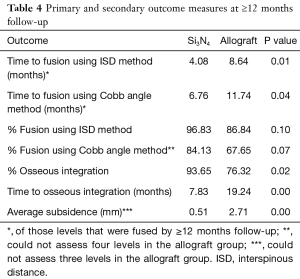
Full table
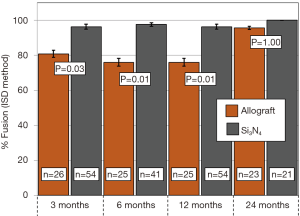
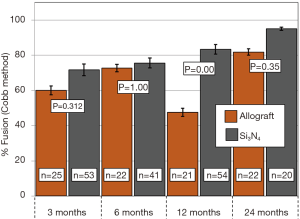
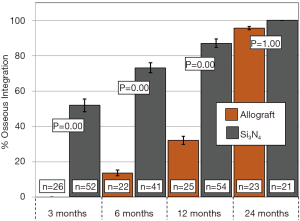
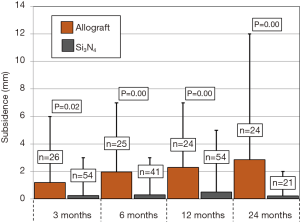
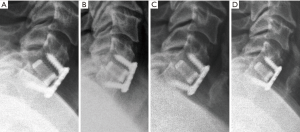
Discussion
For nearly 60 years, ACDF has been the standard of care for patients presenting intractable radiculopathy or myelopathy due to cervical disc herniation, stenosis, spondylosis, and spondylolisthesis (62,63). Historically, autologous bone harvested from the patient’s iliac crest has been utilized to stabilize the operative segment and prevent kyphotic collapse during the fusion process. However, pain associated with the donor site—sometimes lasting up to 12 months post-operatively—has been the major complaint and significant challenge to satisfactory patient outcomes (6,64). To circumvent this persistent morbidity, processed and sterilized cadaver bone began to be used in the 1980s after passage of the National Organ Transplantation Act (65); and thereafter, allograft use rapidly increased while autograft procedures commensurately declined (7). Reviews by Malloy and Hilibrand in 2002 (66) and by Tuchman et al. in 2017 (12) summarized the usage of these two allogenic sources for approximately the past 50 years. Overall, higher arthrodesis rates have been observed with autograft, particularly for earlier fusion and in multi-level procedures. However, for single-level ACDF, patient outcomes appear to be nearly equivalent, and both sources are considered to be effective arthrodesis materials.
Today, the use of both autograft and allograft has been largely supplanted by abiotic cages mostly made from either Ti-alloys or PEEK. Ti-alloys have been around since just after World War II and have been actively used as implants since the 1970’s (26). However, biomedical titanium is essentially bioinert because of a thin passivation layer of titanium dioxide (TiO2) which prevents significant biochemical interactions. Because of its inert nature, surface functionalization of Ti-alloys has been conducted since the 1990s in an effort to enhance the material’s osteoconductivity (67-70). Biomedical PEEK was introduced in the 1990s and rapidly gained acceptance as an intervertebral cage because of its lower cost, favorable modulus, and ease of use (27). Its rise in popularity was accelerated because of subsidence concerns associated with Ti-alloys. However, subsequent studies have shown that the initial and long-term mechanical stability of a spinal implant may be more dependent upon its overall size and geometry rather than its hardness or elastic modulus (54,71,72). Unfortunately, PEEK does not integrate into adjacent host bone and is not visible on plain X-rays (73). In vivo, PEEK spacers heal by the formation of fibrous tissue. This observation has been referred to as the PEEK “halo effect” (74,75). In reality, the hydrophobic nature of PEEK discourages osseointegration by inhibiting protein absorption and cell adhesion on the implant’s surface (41,49,76). Clearly, autograft, allograft, Ti-alloys, and PEEK have their limitations. This has led a number of authors to conclude that the ideal spinal fusion material has yet to be determined (77-79).
In contrast, Si3N4 exhibits many of the important elements of an ideal interbody device. Its inherent chemistry, hydrophilicity, surface charge, and topography are likely responsible for the accelerated fusion observed in this study. Its underlying osteopromotive mechanism involves the release of minute amounts of silicic acid (H4SiO4) and ammonia (NH3). These compounds are known to play active roles in the bone healing process (48,80-83). Eluted ammonia from the ceramic’s surface is also enzymatically converted to nitric oxide (NO) and peroxynitrite (ONOO−) which eventually results in the lysis of adherent bacteria (50,84), Additionally, the anisotropic needle-like Si3N4 grains (<1.0 µm in cross-section and ≤10 µm in length) at the ceramic’s surface have also been shown to play important roles in promoting attachment of proteins and eukaryotic cells while resisting adhesion of prokaryotic cells (47,49,51). In summary, spinal implants produced from Si3N4 appear to have distinct advantages over allograft spacers and other abiotic materials. These benefits include enhanced osteoconductivity (41-48), earlier fusion (56), anti-infective behavior (41,47,49-51,57), and improved radiolucency (39,40).
There are a number of limitations associated with this study. Foremost among them is its retrospective nature. Although the data provide clear statistical evidence of the superiority of Si3N4 cages over allograft spacers, the results presented herein were not compiled using a prospective, randomized, and blinded protocol. The study is also limited from the perspective that the clinical work was conducted only by one surgeon at a single institution.
Conclusions
This retrospective 92-patient ACDF clinical evaluation compared outcomes for traditional allograft spacers and Si3N4 cages over a 24-month follow-up period. The results demonstrated that both materials were effective in achieving acceptable levels of fusion and osseous integration at ≥12 months. However, earlier periods significantly favored the use of the Si3N4 cages. At 3-, 6-, and 12-month follow-ups, the Si3N4 cohort showed faster fusion by both the ISD and Cobb angle methods and greater osseous integration. The Si3N4 group also showed smaller amounts of subsidence at all follow-up periods. At a minimum, the results demonstrate that Si3N4 cages are at least as effective as traditional allograft in ACDF patient outcomes.
Acknowledgements
The authors gratefully acknowledge Clayton Hoffacker of Southern Methodist University who assisted in early data analysis. Appreciation is also given to Professor Giuseppe Pezzotti of the Kyoto Institute of Technology and Dr. Ryan Bock of Amedica Corporation for background information and helpful discussions on analysis of the data and preparation of the manuscript. The Summer Research Fellowship Program at the Indiana University School of Medicine at Fort Wayne, IN is gratefully acknowledged for providing the funding in support of this retrospective study.
Footnote
Conflicts of Interest: Dr. MW Smith is a consulting surgeon to Amedica Corporation. Drs. BJ McEntire and BS Bal are principals and employees of Amedica Corporation which was the manufacturer of the Si3N4 devices used in this study. Dr. DR Romano has no conflicts of interest to declare.
Ethical Statement: Institutional review board (IRB) approval was obtained; but due to the retrospective nature of the study, informed consent by the IRB was not required.
References
- Jacobs W, Willems PC, Kruyt M, et al. Systematic review of anterior interbody fusion techniques for single- and double-level cervical degenerative disc disease. Spine (Phila Pa 1976) 2011;36:E950-60. [Crossref] [PubMed]
- Song KJ, Choi BY. Current concepts of anterior cervical discectomy and fusion: a review of literature. Asian Spine J 2014;8:531-9. [Crossref] [PubMed]
- Buttermann GR. Anterior cervical discectomy and fusion outcomes over 10 years: a prospective study. Spine (Phila Pa 1976) 2018;43:207-14. [Crossref] [PubMed]
- Campana V, Milano G, Pagano E, et al. Bone substitutes in orthopaedic surgery: from basic science to clinical practice. J Mater Sci Mater Med 2014;25:2445-61. [Crossref] [PubMed]
- Kim DH, Rhim R, Li L, et al. Prospective study of iliac crest bone graft harvest site pain and morbidity. Spine J 2009;9:886-92. [Crossref] [PubMed]
- Maharaj MM, Phan K, Mobbs RJ. Anterior cervical discectomy and fusion (ACDF) autograft versus graft substitutes: what do patients prefer?-A clinical study. J Spine Surg 2016;2:105-10. [Crossref] [PubMed]
- McGuire KJ, Harrast J, Herkowitz H, et al. Geographic variation in the surgical treatment of degenerative cervical disc disease: American Board of Orthopedic Surgery Quality Improvement Initiative; part II candidates. Spine (Phila Pa 1976) 2012;37:57-66. [Crossref] [PubMed]
- Phillips R. Spinemarket, Inc. Estimate. 2018. Available online: http://www.thespinemarketgroup.com/
- Young WF, Rosenwasser RH. An early comparative analysis of the use of fibular allograft versus autologous iliac crest graft for interbody fusion after anterior cervical discectomy. Spine (Phila Pa 1976) 1993;18:1123-4. [Crossref] [PubMed]
- Floyd T, Ohnmeiss D. A meta-analysis of autograft versus allograft in anterior cervical fusion. Eur Spine J 2000;9:398-403. [Crossref] [PubMed]
- Suchomel P, Barsa P, Buchvald P, et al. Autologous versus allogenic bone grafts in instrumented anterior cervical discectomy and fusion: a prospective study with respect to bone union pattern. Eur Spine J 2004;13:510-5. [Crossref] [PubMed]
- Tuchman A, Brodke DS, Youssef JA, et al. Autograft versus allograft for cervical spinal fusion: a systematic review. Global Spine J 2017;7:59-70. [Crossref] [PubMed]
- Hacker RJ. A randomized prospective study of an anterior cervical interbody fusion device with a minimum of 2 years of follow-up results. J Neurosurg 2000;93:222-6. [PubMed]
- Thomé C, Krauss JK, Zevgaridis D. A prospective clinical comparison of rectangular titanium cages and iliac crest autografts in anterior cervical discectomy and fusion. Neurosurg Rev 2004;27:34-41. [Crossref] [PubMed]
- Thomé C, Leheta O, Krauss JK, et al. A prospective randomized comparison of rectangular titanium cage fusion and iliac crest autograft fusion in patients undergoing anterior cervical discectomy. J Neurosurg Spine 2006;4:1-9. [Crossref] [PubMed]
- Lind BI, Zoega B, Rosén H. Autograft versus interbody fusion cage without plate fixation in the cervical spine: a randomized clinical study using radiostereometry. Eur Spine J 2007;16:1251-6. [Crossref] [PubMed]
- Lied B, Roenning PA, Sundseth J, et al. Anterior cervical discectomy with fusion in patients with cervical disc degeneration: a prospective outcome study of 258 patients (181 fused with autologous bone graft and 77 fused with a PEEK cage). BMC Surg 2010;10:10. [Crossref] [PubMed]
- Moreland DB, Asch HL, Clabeaux DE, et al. Anterior cervical discectomy and fusion with implantable titanium cage: initial impressions, patient outcomes and comparison to fusion with allograft. Spine J 2004;4:184-91. Erratum in: Spine J 2004;4:following table of contents; discussion 191. [Crossref] [PubMed]
- Yson SC, Sembrano JN, Santos ER. Comparison of allograft and polyetheretherketone (PEEK) cage subsidence rates in anterior cervical discectomy and fusion (ACDF). J Clin Neurosci 2017;38:118-21. [Crossref] [PubMed]
- Cabraja M, Oezdemir S, Koeppen D, et al. Anterior cervical discectomy and fusion: comparison of titanium and polyetheretherketone cages. BMC Musculoskelet Disord 2012;13:172. [Crossref] [PubMed]
- Li ZJ, Wang Y, Xu GJ, et al. Is PEEK cage better than titanium cage in anterior cervical discectomy and fusion surgery? A meta-analysis. BMC Musculoskelet Disord 2016;17:379. [Crossref] [PubMed]
- Seaman S, Kerezoudis P, Bydon M, et al. Titanium vs. polyetheretherketone (PEEK) interbody fusion: Meta-analysis and review of the literature. J Clin Neurosci 2017;44:23-9. [Crossref] [PubMed]
- Ryken TC, Heary RF, Matz PG, et al. Techniques for cervical interbody grafting. J Neurosurg Spine 2009;11:203-20. [Crossref] [PubMed]
- Epstein NE. Iliac crest autograft versus alternative constructs for anterior cervical spine surgery: Pros, cons, and costs. Surg Neurol Int 2012;3:S143-56. [Crossref] [PubMed]
- Miller LE, Block JE. Safety and effectiveness of bone allografts in anterior cervical discectomy and fusion surgery. Spine (Phila Pa 1976) 2011;36:2045-50. [Crossref] [PubMed]
- Wang K. The use of titanium for medical applications in the USA. Mater Sci Eng A 1996;213:134-7. [Crossref]
- Kurtz SM, Devine JN. PEEK biomaterials in trauma, orthopedic, and spinal implants. Biomaterials 2007;28:4845-69. [Crossref] [PubMed]
- Rao PJ, Pelletier MH, Walsh WR, et al. Spine interbody implants: material selection and modification, functionalization and bioactivation of surfaces to improve osseointegration. Orthop Surg 2014;6:81-9. [Crossref] [PubMed]
- Chong E, Pelletier MH, Mobbs RJ, et al. The design evolution of interbody cages in anterior cervical discectomy and fusion: A systematic review. BMC Musculoskelet Disord 2015;16:99. [Crossref] [PubMed]
- Kue R, Sohrabi A, Nagle D, et al. Enhanced proliferation and osteocalcin production by human osteoblast-like MG63 cells on silicon nitride ceramic discs. Biomaterials 1999;20:1195-201. [Crossref] [PubMed]
- Howlett CR, McCartney E, Ching W. The effect of silicon nitride ceramic on rabbit skeletal cells and tissue. An in vitro and in vivo investigation. Clin Orthop Relat Res 1989.293-304. [PubMed]
- Neumann A, Reske T, Held M, et al. Comparative investigation of the biocompatibility of various silicon nitride ceramic qualities in vitro. J Mater Sci Mater Med 2004;15:1135-40. [Crossref] [PubMed]
- Santos C, Ribeiro S, Daguano JK, et al. Development and cytotoxicity evaluation of SiAlONs ceramics. Mater Sci Eng 2007;C27:148-53. [Crossref]
- Neumann A, Kramps M, Ragoß C, et al. Histological and microradiographic appearances of silicon nitride and aluminum oxide in a rabbit femur implantation model. Materwiss Werksttech 2004;35:569-73. [Crossref]
- Guedes e Silva CC, König B Jr, Carbonari MJ, et al. Tissue response around silicon nitride implants in rabbits. J Biomed Mater Res A 2008;84:337-43. [Crossref] [PubMed]
- Guedes e Silva CC, König B, Carbonari MJ, et al. Bone growth around silicon nitride implants—an evaluation by scanning electron microscopy. Mater Charact 2008;59:1339-41. [Crossref]
- McEntire BJ, Lakshminarayanan R, Thirugnanasambandam P, et al. Processing and characterization of silicon nitride bioceramics. Bioceram Dev Appl 2016;6. [Crossref]
- McEntire BJ, Enomoto Y, Zhu W, et al. Surface toughness of silicon nitride bioceramics: II, Comparison with commercial oxide materials. J Mech Behav Biomed Mater 2016;54:346-59. [Crossref] [PubMed]
- Anderson M, Bernero J, Brodke D. Medical imaging characteristics of silicon nitride ceramic a new material for spinal arthroplasty implants. In: 8th Annual Spine Arthroplasty Society Global Symposium on Motion Preservation Technology. Miami, FL; 2008:547.
- Bal BS, Rahaman MN. Orthopedic applications of silicon nitride ceramics. Acta Biomater 2012;8:2889-98. [Crossref] [PubMed]
- Webster TJ, Patel AA, Rahaman MN, et al. Anti-infective and osteointegration properties of silicon nitride, poly(ether ether ketone), and titanium implants. Acta Biomater 2012;8:4447-54. Erratum in: Acta Biomater 2014;10:1485-6. [Crossref] [PubMed]
- Pezzotti G, McEntire BJ, Bock R, et al. Silicon nitride: a synthetic mineral for vertebrate biology. Sci Rep 2016;6:31717. [Crossref] [PubMed]
- Pezzotti G, McEntire BJ, Bock RM, et al. In situ spectroscopic screening of osteosarcoma living cells on stoichiometry-modulated silicon nitride bioceramic surfaces. ACS Biomater Sci Eng 2016;2:1121-34. [Crossref]
- Pezzotti G, Marin E, Adachi T, et al. Bioactive silicon nitride: A new therapeutic material for osteoarthropathy. Sci Rep 2017;7:44848. [Crossref] [PubMed]
- Bock RM, Marin E, Rondinella A, et al. Development of a SiYAlON glaze for improved osteoconductivity of implantable medical devices. J Biomed Mater Res B Appl Biomater 2018;106:1084-96. [Crossref] [PubMed]
- Pezzotti G, Bock RM, Adachi T, et al. Silicon nitride surface chemistry: a potent regulator of mesenchymal progenitor cell activity in bone formation. Appl Mater Today 2017;9:82-95. [Crossref]
- Ishikawa M, de Mesy Bentley KL, McEntire BJ, et al. Surface topography of silicon nitride affects antimicrobial and osseointegrative properties of tibial implants in a murine model. J Biomed Mater Res A 2017;105:3413-21. [Crossref] [PubMed]
- Pezzotti G, Oba N, Zhu W, et al. Human osteoblasts grow transitional Si/N apatite in quickly osteointegrated Si(3)N(4) cervical insert. Acta Biomater 2017;64:411-20. [Crossref] [PubMed]
- Gorth DJ, Puckett S, Ercan B, et al. Decreased bacteria activity on Si3N4 surfaces compared with PEEK or titanium. Int J Nanomedicine 2012;7:4829-40. [PubMed]
- Pezzotti G, Bock RM, McEntire BJ, et al. Silicon nitride bioceramics induce chemically driven lysis in porphyromonas gingivalis. Langmuir 2016;32:3024-35. [Crossref] [PubMed]
- Bock RM, Jones EN, Ray DA, et al. Bacteriostatic behavior of surface modulated silicon nitride in comparison to polyetheretherketone and titanium. J Biomed Mater Res A 2017;105:1521-34. [Crossref] [PubMed]
- Sorrell CC, Hardcastle PH, Druitt RK, et al. Results of 15-Year Clinical Study of Reaction Bonded Silicon Nitride Intervertebral Spacers. Proc 7th World Biomater Conf 2004;1872.
- Youssef JA, Myhre SL, Bal BS. Radiographic Follow-up of Transforaminal Lumbar Fusion with Silicon Nitride Spacers: A Case Report of Two Patients. J Musculoskelet Disord Treat 2016;2:1-8. [Crossref]
- Suh PB, Puttlitz C, Lewis C, et al. The Effect of Cervical Interbody Cage Morphology, Material Composition, and Substrate Density on Cage Subsidence. J Am Acad Orthop Surg 2017;25:160-8. [Crossref] [PubMed]
- Arts MP, Wolfs JFC, Corbin TP. Porous silicon nitride spacers versus PEEK cages for anterior cervical discectomy and fusion: clinical and radiological results of a single-blinded randomized controlled trial. Eur Spine J 2017;26:2372-9. [Crossref] [PubMed]
- Ball HT, McEntire BJ, Bal BS. Accelerated Cervical Fusion of Silicon Nitride versus PEEK Spacers: A Comparative Clinical Study. J Spine 2017;6. [Crossref]
- Rambo WM Jr. Treatment of lumbar discitis using silicon nitride spinal spacers: A case series and literature review. Int J Surg Case Rep 2018;43:61-8. [Crossref] [PubMed]
- Aronson N, Filtzer DL, Bagan M. Anterior cervical fusion by the Smith-Robinson approach. J Neurosurg 1968;29:396-404. [Crossref] [PubMed]
- Karikari IO, Jain D, Owens TR, et al. Impact of subsidence on clinical outcomes and radiographic fusion rates in anterior cervical discectomy and fusion: A systematic review. J Spinal Disord Tech 2014;27:1-10. [Crossref] [PubMed]
- Cannada LK, Scherping SC, Yoo JU, et al. Pseudoarthrosis of the cervical spine: A comparison of radiographic diagnostic measures. Spine (Phila Pa 1976) 2003;28:46-51. [Crossref] [PubMed]
- Kaiser MG, Mummaneni PV, Matz PG, et al. Radiographic assessment of cervical subaxial fusion. J Neurosurg Spine 2009;11:221-7. [Crossref] [PubMed]
- Cloward RB. The anterior approach for removal of ruptured cervical disks. J Neurosurg 1958;15:602-17. [Crossref] [PubMed]
- Cotler JM, Cotler HB, editors. Spinal Fusion Science and Technique. Spinal Fusion Science and Technique. New York: Springer-Verlag, 1990:408.
- Robertson PA, Wray AC. Natural history of posterior iliac crest bone graft donation for spinal surgery: a prospective analysis of morbidity. Spine (Phila Pa 1976) 2001;26:1473-6. [Crossref] [PubMed]
- Boyce T, Edwards J, Scarborough N. Allograft bone. The influence of processing on safety and performance. Orthop Clin North Am 1999;30:571-81. [Crossref] [PubMed]
- Malloy KM, Hilibrand AS. Autograft versus allograft in degenerative cervical disease. Clin Orthop Relat Res 2002.27-38. [Crossref] [PubMed]
- Martin JY, Schwartz Z, Hummert TW, et al. Effect of titanium surface roughness on proliferation, differentiation, and protein synthesis of human osteoblast-like cells (MG63). J Biomed Mater Res 1995;29:389-401. [Crossref] [PubMed]
- Bauer S, Schmuki P, von der Mark K, et al. Engineering Biocompatible Implant Surfaces. Part I: Materials and Surfaces. Prog Mater Sci 2012;58:261-326. [Crossref]
- Lotz EM, Olivares-Navarrete R, Berner S, et al. Osteogenic response of human MSCs and osteoblasts to hydrophilic and hydrophobic nanostructured titanium implant surfaces. J Biomed Mater Res A 2016;104:3137-48. [Crossref] [PubMed]
- Boyan BD, Lotz EM, Schwartz Z. Roughness and Hydrophilicity as Osteogenic Biomimetic Surface Properties. Tissue Eng Part A 2017;23:1479-89. [Crossref] [PubMed]
- Tsantrizos A, Andreou A, Aebi M, et al. Biomechanical stability of five stand-alone anterior lumbar interbody fusion constructs. Eur Spine J 2000;9:14-22. [Crossref] [PubMed]
- Marchi L, Abdala N, Oliveira L, et al. Radiographic and clinical evaluation of cage subsidence after stand-alone lateral interbody fusion. J Neurosurg Spine 2013;19:110-8. [Crossref] [PubMed]
- Kersten RF, van Gaalen SM, de Gast A, et al. Polyetheretherketone (PEEK) cages in cervical applications: a systematic review. Spine J 2015;15:1446-60. [Crossref] [PubMed]
- Toth JM, Wang M, Estes BT, et al. Polyetheretherketone as a biomaterial for spinal applications. Biomaterials 2006;27:324-34. [Crossref] [PubMed]
- Phan K, Hogan JA, Assem Y, et al. PEEK-Halo effect in interbody fusion. J Clin Neurosci 2016;24:138-40. [Crossref] [PubMed]
- Noiset O, Schneider YJ, Marchand-Brynaert J. Fibronectin adsorption or/and covalent grafting on chemically modified PEEK film surfaces. J Biomater Sci Polym Ed 1999;10:657-77. [Crossref] [PubMed]
- Jain S, Eltorai AE, Ruttiman R, et al. Advances in Spinal Interbody Cages. Orthop Surg 2016;8:278-84. [Crossref] [PubMed]
- Boyce T, Lane JM, Boden SD, et al. Basic science symposium I: bone graft substitutes. SAS J 2008;2:55-61. [Crossref] [PubMed]
- Kadam A, Millhouse PW, Kepler CK, et al. Bone substitutes and expanders in Spine Surgery: A review of their fusion efficacies. Int J Spine Surg 2016;10:33. [Crossref] [PubMed]
- Jugdaohsingh R. Silicon and bone health. J Nutr Health Aging 2007;11:99-110. [PubMed]
- Jurkić LM, Cepanec I, Pavelić SK, et al. Biological and therapeutic effects of ortho-silicic acid and some ortho-silicic acid-releasing compounds: New perspectives for therapy. Nutr Metab (Lond) 2013;10:2. [Crossref] [PubMed]
- Evans DM, Ralston SH. Nitric oxide and bone. J Bone Miner Res 1996;11:300-5. [Crossref] [PubMed]
- Saura M, Tarin C, Zaragoza C. Recent insights into the implication of nitric oxide in osteoblast differentiation and proliferation during bone development. ScientificWorldJournal 2010;10:624-32. [Crossref] [PubMed]
- Fang FC. Perspectives series: host/pathogen interactions. Mechanisms of nitric oxide-related antimicrobial activity. J Clin Invest 1997;99:2818-25. [Crossref] [PubMed]