3-dimensional printing for anterior cervical surgery: a review
Introduction
Spondylotic (age-related degenerative changes of the spine) or non-spondylotic lesions that affect the cervical vertebrae can lead to compression of adjacent nervous structures resulting in myelopathic (spinal cord origin) or radiculopathic (spinal nerve origin) symptoms including pain and loss of function (1-4). Non-spondylotic lesions (trauma, tumors, infection and other rarer causes) take up a smaller proportion of myelopathic/radiculopathic causes (1,2), but can result in substantial alteration of the structural anatomy (5-7).
The common mechanism of cervical spondylotic changes include loss of disc height, degeneration of the uncovertebral and facet joints, and disc herniation resulting in spinal nerve encroachment (3). Compression of the nerve root and dorsal root ganglion result in pain (localized neck or may radiate into the upper limbs), which is further aggravated by hypoxia and inflammation (8-11). Osteophyte formation can result from the body coping with flattening of the uncovertebral joints. In addition, ossification of the posterior longitudinal ligament, hypertrophy and calcification of the ligamentum flavum and progressive kyphosis of the cervical spine contributes to stenosis of the central canal, thus ultimately compressing the spinal cord (2,4,12-14). Dynamic compression, particularly during cervical flexion and extension movements, can worsen the pathology (13,15). Chronic cord compression results in a cascade of macro- and micro-changes of the cord leading to a variety of neurological problems that can include: localized neck pain and/or radicular pain into the upper limbs; somatosensory dysfunction of the lower limbs; loss of bladder and bowel control (2,13,15,16). If cord compression is significant, symptoms can correlate with magnetic resonance imaging (MRI) findings indicative of cord signal changes (2,13,16).
In the absence of neurological findings, the recommended approach is non-surgical for pain due to spondylosis (13). However, surgical intervention can significantly improve the outcome of patients if neurological symptoms are present and when imaging clearly demonstrates the involvement of neural structures or when other etiologies are involved (e.g., neoplasms and trauma) (2,13,15,17). The end-goal of surgical intervention is to decompress the neural structures and stabilize pathological segments to prevent movements that can result in further damage, which can be achieved via an anterior or a posterior approach (2,13,18,19). Though there are multiple techniques to achieve these goals, anterior cervical decompression and fusion (ACDF) or anterior cervical corpectomy and fusion (ACCF) with/without posterior stabilization has been shown as an effective treatment option for such cervical spine pathologies (20-22). To date, various ACDF and ACCF implants are available for degenerative changes, but options are more limited for more extreme or distorted anatomies (e.g., traumatic or neoplastic origin). Such extreme pathological anatomy may indicate the design and manufacture of a patient-specific implant (PSI)/custom-made spinal implant.
Since the introduction of three-dimensional printing (3DP) in the 1986 by Hull (23), the manufacturing means have steadily expanded (materials) and improved (precision, reliability) to the point where it is now possible to realize the idea of patient-specific devices. 3DP, also known as Additive Manufacturing or Rapid Prototyping, has been successfully applied in the field of medicine, with orthopedics and neurosurgery being notable early adopters of the technology (24-28). Coupled with advances of medical imaging such as computed tomography (CT) scanning and MRI, accurate (precise and true) 3D models of patient anatomy can be produced. Stereolithographic spinal biomodeling, proposed and adapted by D’Urso et al. in 1999 combined the potential of 3DP with spinal surgery allowing accurate patient-specific spine morphology to be printed in a physical form (29). More recently, 3D printed PSIs have been successfully used to treat patients by Xu et al. in 2016 and Mobbs et al. in 2017 (5,6) (Figure 1). Pre-operative planning, surgical training, intraoperative drill-guides and spinal implants with complex morphology have all been applied with high rates of positive outcomes (24,25,27,28). 3DP applications have been more numerous in the cervical spine, perhaps due to the complex anatomy and the involvement of multiple important structures (vertebral arteries and spinal cord) within a relatively small space, which necessitates higher precision in the engineering of cervical devices (5,30,31).
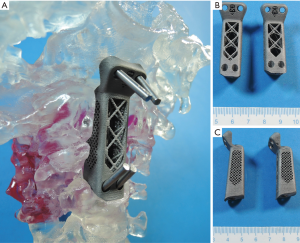
The principle of Additive Manufacturing (3DP) involves layer-by-layer melting and/or fusing of raw printing material(s) to synthesize a 3D part (32-35). Metal (powder), polymers (solid and liquid), ceramics (powder), bio-gels and living cells are currently used as raw materials for 3DP (34,36,37). Models generated by Computer Aided Design (CAD) and computer aided engineering (CAE) are converted into an .STL (STereoLithography) file for 3DP (34,35,38). In terms of spinal bony anatomy, software is used for CT thresholding, segmentation and boundary representation (b-rep) iso-surface model generation (usually via the marching cubes algorithm, or a derivative thereof) to produce an .STL file of the hard or soft tissue anatomical structures of interest (35,39-42). The .stl file is then oriented relative to the build platform and sliced by the build layer thickness to create 2-dimensional tool paths for the 3D printer to perform the process of additive manufacturing (34,35). Currently, various modalities are used to produce 3D printed objects (32,33,35). Depending on the desired outcomes and materials, different methods of 3DP are utilized in the field of neurosurgery with powder bed fusion (electron beam or laser melting or sintering) the most common approach for spinal implants manufactured from Titanium alloys (33,34).
The purpose of this review is to evaluate the current application of 3DP for cervical spinal implants. This includes a review on the available literature on 3D printed PSIs and current available 3D printed “off-the-shelf” (OTS) implants (3D-OTS). Materials suitable for 3DP of spinal implants and the future prospect of cervical implants will be discussed. The review will be concluded with a suggested guide for performing future studies to evaluate the efficacy and safety of 3DP for cervical spinal implants.
Methods
A literature search was performed on four online electronic databases (PubMed, Medline, Web of Science and EMBASE) on the 1st April 2018. To achieve high sensitivity, search terms used were of a combination of: “spine”, “spinal cord”, “cervical”, “neck”, “3-dimensional printing” and “additive manufacturing”. The reference lists of potential studies were screened to identify possible relevant articles. A further search was done online for information [for example white papers with scientific data as well as United States Food and Drug Administration (FDA) 510K approval letters] from spinal implant companies that had recently adopted 3DP as a manufacturing method for implants and received FDA 510(k) clearance for these implants.
Selection criteria
Inclusion criteria
- All literature that reported the use of 3D printed implants for the cervical spine was included;
- For PSIs, anterior cervical fusion implants either corpectomy cages or disc implants are included;
- The implants have to be placed in humans;
- PSIs for all age groups were included;
- Companies with FDA 510(k) clearance for their 3D printed OTS cervical implants were included;
- All cage materials were included.
Exclusion criteria
- PSIs which were not implanted in humans;
- Patient-specific devices such as drill guides were not included;
- 3D printed OTS cervical implants without FDA 510(k) approval were excluded.
There were no review articles that match our study criteria.
Results
Five articles met inclusion criteria (5-7,43). Seventeen patients underwent spinal fusion utilizing 3D printed cervical implants. Three patients with cancer (5-7) and one patient who had spondylotic myelopathy (44) received PSIs. Thirteen other patients with spondylotic myelopathy received pre-planned 3D printed corpectomy implants with 8 different heights (43). A whitepaper from a medical company website [emerging implant technologies (EIT)] (45) reported a patient who underwent two levels ACDF with 3D printed OTS implants. A summary of the articles is provided in Table 1. Table 2 summarizes the FDA 510(k) approval letters for individual cervical implants received by companies that utilizes additive manufacturing (46-50).
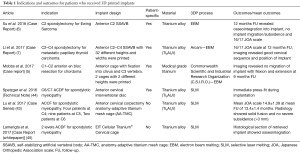
Full table
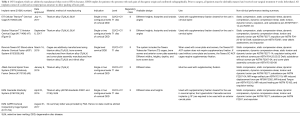
Full table
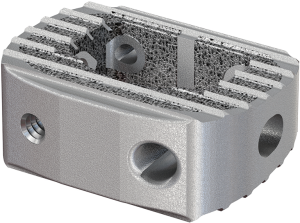
Discussion
Current studies for 3D printed cervical implants
The results of this literature review show the current application of 3DP in manufacturing PSIs for the cervical spine are predominantly due to malignancy (tumor) typically involving the axis (C2 vertebra) (5-7). The axis (C2 vertebra) has a unique anatomy, sitting in the craniocervical junction, allowing the transfer of axial loading from the two lateral masses of the atlas (C1 vertebra) onto the three surfaces (two posterior facets and the anterior vertebral body endplate) of the C3 vertebra (51-53). Malignancies affecting the C2 vertebra are particularly challenging in terms of resection due to their presentation and the unique anatomy of the axis (5-7,53).
The currently available OTS implants for restoration of craniocervical stability post-C2-vertebrectomy requires the use of corpectomy cages, screws and plates (51,52)). However, generic OTS cervical corpectomy cages that are suitable for use in vertebrectomy of levels caudal to C2 may not be as suitable for C2 vertebrectomy, with long term efficacy of such construct remains undetermined. Advancements in 3DP provide a potential solution to this scenario. With the use of 3DP, the unique challenges that the anatomy and functionality of the C2 vertebra poses can potentially be addressed prior to the surgical procedure. All three case reports detailed positive biomechanical outcomes with solid fusion and stability (5-7). The reported intra-operative press-fit for patient specific ACDF cage by Spetzger et al. demonstrates the superiority of the PSI in terms of operating room time efficiency (44). However, there is no follow up data for the outcome of this implant, hence the biomechanical results for this implant remain unknown. The small patient cohort (three) discussed above presents as a potential reporting bias due to small sample size. Further studies involving a larger patient cohorts and long term follow up data are required to assess the benefits/draw backs of 3D printed PSIs.
In a separate study, Zheng et al. compared an integrated artificial axis (IAA), which was additively manufactured to the current available Harms Cage system, in a non-clinical setting. The IAA was manufactured based on a 21-year-old healthy male’s anatomy. The results favored the IAA (53). However, this outcome is limited in terms of external validity as the anatomy of C2 vertebra might vary among individuals. In order to produce a cage suitable for OTS use, population data should be gathered so that normal (and standard shape variation from normal) shape can be defined and used to define the design and size variations of the implants.
The current available 3D printed OTS implants received a class II regulation from the FDA (46-50). This signifies these products must receive a 510(k) letter from the FDA before marketing the implants and the devices are subjected to additional controls. Currently, most of the additively manufactured OTS implants are indicated for ACDFs. The main difference of these devices is the constructs and designs. Since these devices are still new to the market, there is no literature available for short term efficacy and clinical safety of these implants. Lamerigts et al. concluded there is complete osseointegration of the EIT Cellular Titanium® implants (45), however based on the histological image provided in their white paper, there is still a significant abundance of soft and fibrous tissue. Hence, studies for short- and long-term outcomes of these implants should be carried out to evaluate the safety and efficacy of them. Radiographs and CT do not provide the resolution to clearly differentiate osseointegration into 3D porous metal implants.
Materials suitable for 3DP
Our results demonstrate biomedical grade titanium alloy has been the ‘go-to’ material for 3DP of spinal devices. Titanium by itself or in the form of biomedical grade 5 alloy (Ti6Al4V) (not bonded chemically but physically) is an inert metal when oxidized in the TiO2 form that provides excellent biocompatibility and is resistant to corrosion (54). Although pure titanium is inert once oxidized, titanium alloy (Ti6Al4V) has been shown both mechanically and histologically to bond directly with bone under static condition after being implanted for some time (55). The high porosity and interconnectivity of 3D printed titanium lattices potentially serves as a good platform for bone and tissue on and in-growth (54,56). The use of Ti6Al4V in 3DP via advanced powder manufacturing routes to create implants for both in vivo and in vitro testing has shown the biocompatibility and osseointegration potential of Ti6Al4V (56,57). Radiological fusion as observed clinically from recent use of Ti6Al4V in 3DP for spinal implants further confirms the suitability and compatibility of Ti6Al4V (58). However, there are a few drawbacks in the use of titanium for spinal implants. The high elastic modulus and stiffness of titanium (110 GPa) compared to cortical (3–30 GPa) and cancellous bone (0.02–2 GPa) serves as a high potential for subsidence (59-61). Titanium itself is a metal which has high radiodensity, making fusion assessment difficult in static radiographic imaging (CT and planar X-ray). Although it is electromagnetically inert, titanium can still cause imaging artefact/flaring in MRIs again making the images difficult to interpret around the implant (62).
Currently other materials such as PolyEther-Ether-Ketone (PEEK) and silicon nitride (Si3N4) have been used to manufacture OTS cervical implants but have not yet been used for 3DP of spinal implants. A major benefit for PEEK is its radiolucency and biocompatibility (63,64) making it a common material for spinal implants. Having an elastic modulus similar or less than cortical bone is often proposed to reduce the risk of subsidence (65), although recent mechanical testing using the ASTM subsidence test by Suh et al. has shown that this may not be the case (61). However, there is a potential for suboptimal osseointegration for PEEK implants as reported by Phan et al., demonstrating a “PEEK-Halo” effect seen on CT (65). Currently, various techniques are being trialed to improve osseointegration on PEEK implants including coating the surfaces of the implants with a plasma sprayed coating of titanium forming a Ti/PEEK combined cage (66,67). Early clinical data suggest good radiological fusion of such devices (68,69) whilst the risk of delamination of the plasma sprayed coating exists either on implantation or in life service. Incorporating a thin layer of titanium into the PEEK itself is another technique used to provide a titanium interface for biological on growth (70). NanoMetalene (NM) describes the commercial application of this process on spinal interbody implants where a sub-micron layer of commercially pure titanium is molecularly bonded to a PEEK implant using a proprietary, high-energy, low-temperature process that differs from other coating applications and maximizes implant surface area with titanium nanotopography (70).
On the other hand, the inert chemistry of Si3N4 serves as a potential candidate for spinal implants (71). Several studies have demonstrated superiority in bacteriostatic behavior of Si3N4 compared to PEEK and titanium implants (72-74). Additionally, Si3N4 was shown to promote bone growth and fusion (72,75), but there is currently limited literature discussing the outcomes of Si3N4 spinal implants.
Both PEEK and Si3N4 serve as a potential candidate for 3D printed spinal implants. 3DP of ceramics has advanced to allow the manufacturing of cellular structure which serves as a potential surface for bone in-growth (76,77). With future advances in 3DP technology, materials could potentially be combined to form an implant which serves all three purposes of promoting osseointegration, minimizing subsidence potential, whilst maintaining sterility.
Subsidence and osseointegration
Subsidence is a common phenomenon with the use of spinal implants. Since its inception, 3DP allows the fabrication of varying surfaces and structures. This benefit of 3DP as a manufacturing method allows complex structures to be printed such as lattices. This allows medical devices to feature ‘in growth’ topologies and pores that are specifically designed to encourage osseointegration and greater bone bonding strength to the device (59,78). For instance, 3DP of titanium allows the manufacture of open cellular Ti6Al4V lattices with different densities to achieve different elastic moduli, again with the aim of matching the stiffness of spinal bone (79,80). Solid titanium has a very high elastic modulus compared to cortical bone; 110 GPa compared to 3–30 GPa respectively. This differential in stiffness between device material and bone can instigate local bone remodeling leading to ‘stress shielding’. More extreme inflammatory responses and remodeling caused by bone damage (fracture and/or micro fracture) increase the risk of subsidence, bone atrophy and implant failure (54,59,81,82). Device stiffness alone is not responsible for subsidence as although having an elastic modulus lower than cortical bone, subsidence was observed in the use of standalone PEEK cages for ACDFs (83). Some authors argued that subsidence is an inevitable radiological finding but beneficial for fusion in terms of osseointegration (84). Utilizing 3DP, there is the potential of achieving a “sweet spot” to promote osseointegration and reduce the chances of subsidence by altering the porosity of an implant to achieve a lower elastic modulus (78,79). However, this can potentially result in implant failure as highly porous, less stiff implants will be more prone to fatigue failure. Another important factor for the adaptation of PSIs is to increase the contact area between implant and the endplates as reported by Spetzger et al. and Mobbs et al. (44,85). The aim of this is to achieve even load distribution along the implant surface thereby reducing the chance of subsidence (86).
Pros and cons of PSIs
The main benefit for PSIs compared to OTS implants (3DP or traditionally manufactured) are that PSIs are designed based on the patient’s anatomy, surgical and biomechanical requirements. The specificity of PSIs reduces the need of excessive removal of surrounding structures, thereby preserving the anatomy of the patient during implantation. This may lead to shortening of operative time, preservation of anatomy which requires lesser dissection, direct press-fit of implant and improved stability of construct (85). In the cervical spine, the main goal of PSIs is to achieve stability and restoring functionality especially for unique anatomies such as the axis (5-7). The rationale of utilizing PSIs as opposed to OTS implants allows complex anatomical morphology to be accommodated and unique features such as pre-planned screw trajectories to be added, thus improving intra-operative accuracy for screw placement (58) which may have some advantages in specific cases.
However, drawbacks remain with PSIs as they require meticulous planning and design before the implant can be manufactured (44,87,88). The process leads to the increase in requirements for specialized personnel such as biomedical engineers with a good knowledge of CAD, anatomy and surgical procedures. The printing itself requires high-end 3D printers to achieve suitable precision and specialized materials which are not yet widely available, the need for post-processing of the printed implants as well as final cleaning and packaging. The possibility of lack of fit at the time of surgical procedure also remains. Hence, the availability, time and cost remain a drawback for the wide use of PSIs. Long-term studies are also unavailable at the moment to evaluate the efficacy and safety of both 3D printed patient-specific and OTS implants.
Future prospect
With the advancement of technology, 3DP as a manufacturing method will likely become increasingly available and cheaper. This will promote the use of PSIs in the future. The time taken and cost of 3DP PSIs will also reflect advancement in speed of imaging segmentation and device design. New materials that are biocompatible, radiolucent and promote bone on/in-growth will likely replace the current materials such as Ti6Al4V and PEEK. Stem cells from patients may be incorporated into 3D printed implants to promote healing (89) and osseointegration. 3D printers may also be widely available in hospitals to allow immediate printing of PSIs when required. Coupled with advancements of preoperative imaging and segmentation, robotics and intra-operative image-based navigation, surgical outcomes for patients receiving 3D printed implants and organs will become faster, more cost effective, safer and less invasive.
Future studies
3DP is still in the early phase in terms of cervical spinal implants. The current sparsity of available literature limits evaluation regarding the safety and efficacy of this technology. With cost and availability being the major hindrance, studies with higher of level of evidence such as randomized controlled trials cannot yet be carried out. However, there is a sparsity of reports currently available in the literature, necessitating short and long-term outcome studies for both 3D printed patient-specific and OTS implants to be carried out. The studies ideally should include quantitative and qualitative data to assess the outcomes of these implants, for example: pre- and post-operation clinical scores [Japanese Orthopedic Association (JOA) score, Neck Disability Index (NDI) and Visual Analog Scale (VAS) for arm and neck]; (Cobb’s) angles; degree of distraction; rate of subsidence; implant migration and post-operative complications. Other biocompatible materials that may reduce subsidence and promote osseointegration and healing can also be trialed utilizing additive manufacturing. This can include combining different materials to achieve a superior outcome than standalone materials.
Conclusions
Although 3DP is still in the early stages of development for cervical reconstructive surgery, there is no doubt of the versatility of this technology for personalization of implants and management of complex anatomical deformities. The current sparsity of available literature limits the evaluation regarding the safety and efficacy of this technology, especially with regards to OTS implants where there is currently no documented clinical benefit as compared with OTS implants produced via subtractive manufacturing techniques.
Cost and speed of access to personalized implants remains a major hindrance to their wide spread adoption, with no studies including higher levels of evidence (such as randomized controlled trials) available. Due to the shortcomings in the literature, we urge that appropriate short and long-term outcome studies for both additively manufactured PSIs and OTS implants to be performed, with quantitative and qualitative data to assess the outcomes. Further works on biocompatible materials utilizing additive manufacturing require investigation, especially as Titanium artefact in postoperative imaging remains an issue to radiographically assess the fusion status.
Acknowledgements
None.
Footnote
Conflicts of Interest: The authors have no conflicts of interest to declare.
References
- Shelerud RA, Paynter KS. Rarer causes of radiculopathy: spinal tumors, infections, and other unusual causes. Phys Med Rehabil Clin N Am 2002;13:645-96. [Crossref] [PubMed]
- Tetreault L, Goldstein CL, Arnold P, et al. Degenerative Cervical Myelopathy: A Spectrum of Related Disorders Affecting the Aging Spine. Neurosurgery 2015;77 Suppl 4:S51-67. [Crossref] [PubMed]
- Carette S, Fehlings MG. Cervical Radiculopathy. N Engl J Med 2005;353:392-9. [Crossref] [PubMed]
- Baptiste DC, Fehlings MG. Pathophysiology of cervical myelopathy. Spine J 2006;6:190S-197S. [Crossref] [PubMed]
- Mobbs RJ, Coughlan M, Thompson R, et al. The utility of 3D printing for surgical planning and patient-specific implant design for complex spinal pathologies: case report. J Neurosurg Spine 2017;26:513-8. [Crossref] [PubMed]
- Xu N, Wei F, Liu X, et al. Reconstruction of the Upper Cervical Spine Using a Personalized 3D-Printed Vertebral Body in an Adolescent With Ewing Sarcoma. Spine 2016;41:E50-4. [Crossref] [PubMed]
- Li X, Wang Y, Zhao Y, et al. Multilevel 3D Printing Implant for Reconstructing Cervical Spine With Metastatic Papillary Thyroid Carcinoma. Spine 2017;42:E1326-E1330. [Crossref] [PubMed]
- Howe JF, Loeser JD, Calvin WH. Mechanosensitivity of dorsal root ganglia and chronically injured axons: a physiological basis for the radicular pain of nerve root compression. Pain 1977;3:25-41. [Crossref] [PubMed]
- Song XJ, Hu SJ, Greenquist KW, et al. Mechanical and thermal hyperalgesia and ectopic neuronal discharge after chronic compression of dorsal root ganglia. J Neurophysiol 1999;82:3347-58. [Crossref] [PubMed]
- Sugawara O, Atsuta Y, Iwahara T, et al. The effects of mechanical compression and hypoxia on nerve root and dorsal root ganglia. An analysis of ectopic firing using an in vitro model. Spine 1996;21:2089-94. [Crossref] [PubMed]
- Kang JD, Georgescu HI, McIntyre-Larkin L, et al. Herniated cervical intervertebral discs spontaneously produce matrix metalloproteinases, nitric oxide, interleukin-6, and prostaglandin E2. Spine 1995;20:2373-8. [Crossref] [PubMed]
- Galbusera F, van Rijsbergen M, Ito K, et al. Ageing and degenerative changes of the intervertebral disc and their impact on spinal flexibility. Eur Spine J 2014;23 Suppl 3:S324-32. [PubMed]
- Tracy JA, Bartleson JD. Cervical Spondylotic Myelopathy. Neurologist 2010;16:176-87. [Crossref] [PubMed]
- Inamasu J, Guiot BH, Sachs DC. Ossification of the posterior longitudinal ligament: an update on its biology, epidemiology, and natural history. Neurosurgery 2006;58:1027-39; discussion 1039. [Crossref] [PubMed]
- Karadimas SK, Erwin WM, Ely CG, et al. Pathophysiology and natural history of cervical spondylotic myelopathy. Spine 2013;38:S21-36. [Crossref] [PubMed]
- Kalsi-Ryan S, Karadimas SK, Fehlings MG. Cervical spondylotic myelopathy: the clinical phenomenon and the current pathobiology of an increasingly prevalent and devastating disorder. Neuroscientist 2013;19:409-21. [Crossref] [PubMed]
- Davarski AN, Kitov BD, Zhelyazkov CB, et al. Surgical management of metastatic tumors of the cervical spine. Folia Med (Plovdiv) 2013;55:39-45. [Crossref] [PubMed]
- Lawrence BD, Shamji MF, Traynelis VC, et al. Surgical management of degenerative cervical myelopathy: a consensus statement. Spine 2013;38:S171-2. [Crossref] [PubMed]
- Rao RD, Gourab K, David KS. Operative treatment of cervical spondylotic myelopathy. J Bone Joint Surg Am 2006;88:1619-40. [Crossref] [PubMed]
- Maharaj MM, Mobbs RJ, Hogan J, et al. Anterior cervical disc arthroplasty (ACDA) versus anterior cervical discectomy and fusion (ACDF): a systematic review and meta-analysis. J Spine Surg 2015;1:72-85. [PubMed]
- Wang T, Wang H, Liu S, et al. Anterior cervical discectomy and fusion versus anterior cervical corpectomy and fusion in multilevel cervical spondylotic myelopathy: A meta-analysis. Medicine 2016;95:e5437. [Crossref] [PubMed]
- Wen ZQ, Du JY, Ling ZH, et al. Anterior cervical discectomy and fusion versus anterior cervical corpectomy and fusion in the treatment of multilevel cervical spondylotic myelopathy: systematic review and a meta-analysis. Ther Clin Risk Manag 2015;11:161-70. [PubMed]
- Hull CW, inventor; UVP, Inc., San Gabriel, California, assignee. Apparatus for production of three-dimensional objects by stereolithography. United States patent US 4,575,330 A 1986.
- Ploch CC, Mansi C, Jayamohan J, et al. Using 3D Printing to Create Personalized Brain Models for Neurosurgical Training and Preoperative Planning. World Neurosurg 2016;90:668-74. [Crossref] [PubMed]
- Randazzo M, Pisapia JM, Singh N, et al. 3D printing in neurosurgery: A systematic review. Surg Neurol Int 2016;7:S801-S809. [Crossref] [PubMed]
- Eltorai AE, Nguyen E, Daniels AH. Three-Dimensional Printing in Orthopedic Surgery. Orthopedics 2015;38:684-7. [Crossref] [PubMed]
- Tack P, Victor J, Gemmel P, et al. 3D-printing techniques in a medical setting: a systematic literature review. Biomed Eng Online 2016;15:115. [Crossref] [PubMed]
- Wilcox B, Mobbs RJ, Wu AM, et al. Systematic review of 3D printing in spinal surgery: the current state of play. J Spine Surg 2017;3:433-43. [Crossref] [PubMed]
- D'Urso PS, Askin G, Earwaker JS, et al. Spinal biomodeling. Spine 1999;24:1247-51. [Crossref] [PubMed]
- Gao F, Wang Q, Liu C, et al. Individualized 3D printed model-assisted posterior screw fixation for the treatment of craniovertebral junction abnormality: a retrospective study. J Neurosurg Spine 2017;27:29-34. [Crossref] [PubMed]
- Zhang G, Yu Z, Chen X, et al. Accurate placement of cervical pedicle screws using 3D-printed navigational templates: An improved technique with continuous image registration. Orthopade 2018;47:428-36. [Crossref] [PubMed]
- Mitsouras D, Liacouras PC. 3D Printing Technologies. In: Rybicki FJ, Grant GT. editors. 3D Printing in Medicine: A Practical Guide for Medical Professionals 2017:5-22.
- Dutta B, Froes FH. The Additive Manufacturing (AM) of titanium alloys. Metal Powder Report 2017;72:96-106. [Crossref]
- Ventola CL. Medical Applications for 3D Printing: Current and Projected Uses. P T 2014;39:704-11. [PubMed]
- Gross BC, Erkal JL, Lockwood SY, et al. Evaluation of 3D Printing and Its Potential Impact on Biotechnology and the Chemical Sciences. Anal Chem 2014;86:3240-53. [Crossref] [PubMed]
- Schubert C, van Langeveld MC, Donoso LA. Innovations in 3D printing: a 3D overview from optics to organs. Br J Ophthalmol 2014;98:159-61. [Crossref] [PubMed]
- Gu Q, Hao J, Lu Y, et al. Three-dimensional bio-printing. Sci China Life Sci 2015;58:411-9. [Crossref] [PubMed]
- Iancu C, Iancu D, Stăncioiu A. From cad model to 3D print via "stl" file format. Fiability & Durability/Fiabilitate si Durabilitate 2010;(1):73-80.
- Wroe S, Parr WCH, Ledogar JA, et al. Computer simulations show that Neanderthal facial morphology represents adaptation to cold and high energy demands, but not heavy biting. Proc Biol Sci 2018;285. [PubMed]
- Parr WC, Chamoli U, Jones A, et al. Finite element micro-modelling of a human ankle bone reveals the importance of the trabecular network to mechanical performance: New methods for the generation and comparison of 3D models. J Biomech 2013;46:200-5. [Crossref] [PubMed]
- Parr WC, Wilson LAB, Wroe S, et al. Cranial Shape and the Modularity of Hybridization in Dingoes and Dogs; Hybridization Does Not Spell the End for Native Morphology. Evolutionary Biology 2016;43:171-87. [Crossref]
- Tan CJ, Parr WCH, Walsh WR, et al. Influence of Scan Resolution, Thresholding, and Reconstruction Algorithm on Computed Tomography-Based Kinematic Measurements. J Biomech Eng 2017;139. [Crossref] [PubMed]
- Lu T, Liu C, Yang B, et al. Single-Level Anterior Cervical Corpectomy and Fusion Using a New 3D-Printed Anatomy-Adaptive Titanium Mesh Cage for Treatment of Cervical Spondylotic Myelopathy and Ossification of the Posterior Longitudinal Ligament: A Retrospective Case Series Study. Med Sci Monit 2017;23:3105-14. [Crossref] [PubMed]
- Spetzger U, Frasca M, Konig SA. Surgical planning, manufacturing and implantation of an individualized cervical fusion titanium cage using patient-specific data. Eur Spine J 2016;25:2239-46. [Crossref] [PubMed]
- Lamerigts N, Brink WVD. Complete osseointegration of a retrieved 3D printed porous titanium cervical implant - a case report (whitepaper) 2017.
- Department of Health & Human Services FDA. 510(k) Approval Letter for K170503, EIT Cellular Titanium® Cervical Cage, EIT Cellular Titanium® PLIF Cages, EIT Cellular Titanium® TLIF Cages, and EIT Cellular Titanium® ALIF Cages. In: Department of Health & Human Services FaDA, editor 2017.
- Department of Health & Human Services FDA. 510(k) Approval Letter for K171496, Stryker Tritanium® C Anterior Cervical Cage. In: Department of Health & Human Services FDA, editor 2017.
- Department of Health & Human Services FDA. 510(k) Approval Letter for K153250, Renovis Tesera SC Stand-alone Anterior Cervical Fusion (ACF) System. In: Department of Health & Human Services FDA, editor 2016.
- Department of Health & Human Services FDA. 510(k) Approval Letter for K173159, 4Web Cervical Spinal Truss System (CSTS) Interbody Fusion Device. In: Department of Health & Human Services FDA, editor 2018.
- Department of Health & Human Services FDA. 510(k) Approval Letter for K160125, K2M Cascadia Interbody System. In: Department of Health & Human Services FDA, editor 2016.
- Jeszenszky D, Fekete TF, Melcher R, et al. C2 prosthesis: anterior upper cervical fixation device to reconstruct the second cervical vertebra. Eur Spine J 2007;16:1695-700. [Crossref] [PubMed]
- Puttlitz CM, Harms J, Xu Z, et al. A biomechanical analysis of C2 corpectomy constructs. Spine J 2007;7:210-5. [Crossref] [PubMed]
- Zheng Y, Wang J, Liao S, et al. Biomechanical evaluation of a novel integrated artificial axis: A finite element study. Medicine 2017;96:e8597. [Crossref] [PubMed]
- Rao PJ, Pelletier MH, Walsh WR, et al. Spine Interbody Implants: Material Selection and Modification, Functionalization and Bioactivation of Surfaces to Improve Osseointegration. Orthop Surg 2014;6:81-9. [Crossref] [PubMed]
- Takatsuka K, Yamamuro T, Nakamura T, et al. Bone-bonding behavior of titanium alloy evaluated mechanically with detaching failure load. J Biomed Mater Res 1995;29:157-63. [Crossref] [PubMed]
- Li JP, Li SH, Van Blitterswijk CA, et al. A novel porous Ti6Al4V: characterization and cell attachment. J Biomed Mater Res A 2005;73:223-33. [Crossref] [PubMed]
- Sidambe AT. Biocompatibility of Advanced Manufactured Titanium Implants—A Review. Materials 2014;7:8168-88. [Crossref] [PubMed]
- Choy WJ, Mobbs RJ, Wilcox B, et al. Reconstruction of Thoracic Spine Using a Personalized 3D-Printed Vertebral Body in Adolescent with T9 Primary Bone Tumor. World Neurosurg 2017;105:1032.e13-1032.e17. [Crossref] [PubMed]
- Wang X, Xu S, Zhou S, et al. Topological design and additive manufacturing of porous metals for bone scaffolds and orthopaedic implants: A review. Biomaterials 2016;83:127-41. [Crossref] [PubMed]
- Rho JY, Ashman RB, Turner CH. Young's modulus of trabecular and cortical bone material: ultrasonic and microtensile measurements. J Biomech 1993;26:111-9. [Crossref] [PubMed]
- Suh PB, Puttlitz C, Lewis C, et al. The Effect of Cervical Interbody Cage Morphology, Material Composition, and Substrate Density on Cage Subsidence. J Am Acad Orthop Surg 2017;25:160-8. [Crossref] [PubMed]
- Ernstberger T, Heidrich G, Bruening T, et al. The interobserver-validated relevance of intervertebral spacer materials in MRI artifacting. Eur Spine J 2007;16:179-85. [Crossref] [PubMed]
- Rivard CH, Rhalmi S, Coillard C. In vivo biocompatibility testing of peek polymer for a spinal implant system: A study in rabbits. J Biomed Mater Res 2002;62:488-98. [Crossref] [PubMed]
- Wenz LM, Merritt K, Brown SA, et al. In vitro biocompatibility of polyetheretherketone and polysulfone composites. J Biomed Mater Res 1990;24:207-15. [Crossref] [PubMed]
- Phan K, Hogan JA, Assem Y, et al. PEEK-Halo effect in interbody fusion. J Clin Neurosci 2016;24:138-40. [Crossref] [PubMed]
- Kotsias A, Mularski S, Kühn B, et al. Does partial coating with titanium improve the radiographic fusion rate of empty PEEK cages in cervical spine surgery? A comparative analysis of clinical data. Patient Saf Surg 2017;11:13. [Crossref] [PubMed]
- Chong E, Mobbs RJ, Pelletier MH, et al. Titanium/Polyetheretherketone Cages for Cervical Arthrodesis with Degenerative and Traumatic Pathologies: Early Clinical Outcomes and Fusion Rates. Orthop Surg 2016;8:19-26. [Crossref] [PubMed]
- Assem Y, Mobbs RJ, Pelletier MH, et al. Radiological and clinical outcomes of novel Ti/PEEK combined spinal fusion cages: a systematic review and preclinical evaluation. Eur Spine J 2017;26:593-605. [Crossref] [PubMed]
- Mobbs RJ, Phan K, Assem Y, et al. Combination Ti/PEEK ALIF cage for anterior lumbar interbody fusion: Early clinical and radiological results. J Clin Neurosci 2016;34:94-9. [Crossref] [PubMed]
- Walsh WR, Pelletier MH, Christou C, et al. The in vivo response to a novel Ti coating compared with polyether ether ketone: evaluation of the periphery and inner surfaces of an implant. Spine J 2018;18:1231-40. [Crossref] [PubMed]
- Bock RM, McEntire BJ, Bal BS, et al. Surface modulation of silicon nitride ceramics for orthopaedic applications. Acta Biomater 2015;26:318-30. [Crossref] [PubMed]
- Webster TJ, Patel AA, Rahaman MN, et al. Anti-infective and osteointegration properties of silicon nitride, poly(ether ether ketone), and titanium implants. Acta Biomater 2012;8:4447-54. [Crossref] [PubMed]
- Gorth DJ, Puckett S, Ercan B, et al. Decreased bacteria activity on Si(3)N(4) surfaces compared with PEEK or titanium. Int J Nanomedicine 2012;7:4829-40. [PubMed]
- Bock RM, Jones EN, Ray DA, et al. Bacteriostatic behavior of surface modulated silicon nitride in comparison to polyetheretherketone and titanium. J Biomed Mater Res A 2017;105:1521-34. [Crossref] [PubMed]
- Bal BS, Rahaman MN. Orthopedic applications of silicon nitride ceramics. Acta Biomater 2012;8:2889-98. [Crossref] [PubMed]
- Felzmann R, Gruber S, Mitteramskogler G, et al. Lithography‐Based Additive Manufacturing of Cellular Ceramic Structures. Advanced Engineering Materials 2012;14:1052-8. [Crossref]
- Melchels FP, Domingos MA, Klein TJ, et al. Additive manufacturing of tissues and organs. Progress in Polymer Science 2012;37:1079-104. [Crossref]
- Murr LE, Gaytan SM, Medina F, et al. Next-generation biomedical implants using additive manufacturing of complex, cellular and functional mesh arrays. Philos Trans A Math Phys Eng Sci 2010;368:1999-2032. [PubMed]
- Murr LE, Gaytan SM, Medina F, et al. Characterization of Ti–6Al–4V open cellular foams fabricated by additive manufacturing using electron beam melting. Materials Science and Engineering: A 2010;527:1861-8. [Crossref]
- Heary RF, Parvathreddy N, Sampath S, et al. Elastic modulus in the selection of interbody implants. J Spine Surg 2017;3:163-7. [Crossref] [PubMed]
- Niu CC, Liao JC, Chen WJ, et al. Outcomes of Interbody Fusion Cages Used in 1 and 2-levels Anterior Cervical Discectomy and Fusion: Titanium Cages Versus Polyetheretherketone (PEEK) Cages. J Spinal Disord Tech 2010;23:310-6. [Crossref] [PubMed]
- Skinner HB. Composite technology for total hip arthroplasty. Clin Orthop Relat Res 1988.224-36. [PubMed]
- Kao TH, Wu CH, Chou YC, et al. Risk factors for subsidence in anterior cervical fusion with stand-alone polyetheretherketone (PEEK) cages: a review of 82 cases and 182 levels. Arch Orthop Trauma Surg 2014;134:1343-51. [Crossref] [PubMed]
- Kim YS, Park JY, Moon BJ, et al. Is stand alone PEEK cage the gold standard in multilevel anterior cervical discectomy and fusion (ACDF)? Results of a minimum 1-year follow up. J Clin Neurosci 2018;47:341-6. [Crossref] [PubMed]
- Mobbs RJ, Choy WJ, Wilson P, et al. L5 En-Bloc Vertebrectomy with Customized Reconstructive Implant: Comparison of Patient-Specific Versus Off-the-Shelf Implant. World Neurosurg 2018;112:94-100. [Crossref] [PubMed]
- Suh PB, Lewis C, Puttlitz CM, et al. The Influence of Vertebral Endplate Density, Cage Contact Area and Cage Modulus on the Incidence of Interbody Cage Subsidence. Spine J 2015;10:S178. [Crossref]
- D'Urso PS, Williamson OD, Thompson RG. Biomodeling as an aid to spinal instrumentation. Spine 2005;30:2841-5. [Crossref] [PubMed]
- Izatt MT, Thorpe PL, Thompson RG, et al. The use of physical biomodelling in complex spinal surgery. Eur Spine J 2007;16:1507-18. [Crossref] [PubMed]
- Di Bella C, Fosang A, Donati DM, et al. 3D Bioprinting of Cartilage for Orthopedic Surgeons: Reading between the Lines. Front Surg 2015;2:39. [Crossref] [PubMed]