Orthobiologics in minimally invasive lumbar fusion
Introduction
Like many other surgical subspecialties, the field of spine surgery has undergone a steady shift toward minimally invasive (MI) procedures. While traditional open and MI approaches to spinal fusion have similar success rates, MI techniques offer distinct advantages to patients. MI spine surgery can result in decreased intraoperative blood loss, shorter length of stay, and a reduction in the use of postoperative opioids (1-3). Additionally, patients are less likely to be readmitted with complications and have improved cosmetic outcomes due to smaller incision size (2).
While in 2010 MI spine procedures accounted for 1/6 of all spine surgeries performed in the United States, this figure is expected to increase to more than 1/2 by 2020 (4). Although some clinical studies demonstrate equivalent or superior outcomes following MI lumbar fusion in comparison to open procedures, the concern for nonunion persists. For example, MI techniques are associated with limited exposure, reducing the surface area preparation which can lead to high fusion rates in an open setting (5). As surgeon and patient preference for MI procedures increases, selecting the appropriate implant material will remain critically important in spine surgery. Here, we review the various biologic and biomimetic implants available for use in in this clinical setting.
Biologic materials
In order to stimulate new bone growth between two vertebral bodies, the surgeon must select an implant with adequate osteoconductive, osteoinductive, and/or osteogenic properties. Orthobiologic implants, such as bone grafts, stem cells, and proteins, possess some or all of these properties, and are used to create an environment favorable for new bone formation and successful fusion.
Autologous bone
Autologous grafts used in spine surgery are commonly derived from cancellous and non-vascularized cortical bone. Cancellous bone is characterized by its trabecular structure and high concentration of osteoblasts and osteocytes (6). Although cancellous bone lacks structural integrity, its porosity facilitates neovascularization, cellular recruitment, osteoid deposition, and ultimate mineralization and resorption of the graft material (6). In contrast to cancellous bone, cortical bone grafts offer greater mechanical stability; however, its tightly organized, dense structure does not permit substantial vascularization, and contains relatively few osteogenic cells. Incorporation of cortical bone grafts occurs slowly via creeping substitution, a process in which new bone formation and graft resorption occur simultaneously. Ultimately, once healing occurs, there is minimal to no residual weakness (7).
Iliac crest bone graft (ICBG)
Due to its consistent osteoconductive, osteoinductive, and osteogenic properties, ICBG has long been considered the gold standard material used to achieve spinal fusion (8). In addition to its use in traditional open procedures, autograft bone may be similarly employed in MI spine fusions. In a review of biologic materials utilized in MI spine fusion procedures, studies utilizing ICBG alone achieved a 96% rate of fusion overall (9). While ICBG can be used to reliably achieve fusion, graft harvest is associated with significant morbidity, including pain, nerve injury, hematoma, and increased rates of infection (10).
In an effort to reduce donor site morbidity, Lopez et al. outlined a novel surgical technique for MI ICBG harvest for use in conjunction with MI transforaminal lumbar interbody fusion (MIS-TLIF) (11). This method allows for collection of sufficient autograft (25–50 cm3) using a tubular retractor, core reamer, and curved curettes through the same incision as the surgical site (11). Haws et al. utilized this harvesting technique in a comparison of patient outcomes following MIS-TLIF using ICBG alone versus BMP-2 plus autograft or allograft (12). Although the total operative time and estimated blood loss were significantly greater in the ICBG group than the BMP-2 group, these increases did not affect the overall length of hospital stay. Furthermore, patients in the BMP-2 cohort required greater hourly narcotic consumption for adequate pain control on postoperative day two (P=0.0013), and incurred significantly greater overall hospital costs ($21,645 vs. $19,315, P<0.001) than those in the autograft group (12).
Local bone graft
In addition to ICBG, bone graft may be harvested from spinous processes, lamina, and facets in the surgical field. This can be completed in both open and MI procedures and, like other autologous tissues, local bone graft benefits from a lack of immunogenicity and risk of disease transmission. Locally harvested bone may be used as an alternative to ICBG to avoid additional donor site morbidity. However, the limited volume of bone available during the procedure precludes its use as the sole graft material in multilevel fusions (13).
In MI lumbar surgeries, the rate of radiographic fusion achieved using local autograft alone ranges from 61–100% (9). Careful bone preparation, including morcelization and thorough soft tissue removal, is thought to increase the chance of fusion and favorable clinical outcome (14). When used in combination with ICBG in posterior approaches to MI spine fusion, local autograft has been shown to perform less effectively, achieving rates of fusion between 83% and 91%. These findings may be explained by in vitro evidence which suggests that harvest location may dictate the osteogenic potential of bone grafts. It has been shown that the osteoblast content of the cancellous bone obtained via laminectomy is greater than that of bone obtained from the iliac crest (15).
Bone marrow aspirate (BMA)
BMA contains pluripotent mesenchymal stem cells (MSCs) that can be directed to differentiate into osteogenic progenitor cells in the appropriate environment. These cells are easily isolated and amenable to “ex vivo” expansion, allowing preservation for later therapeutic application. Additionally, naïve undifferentiated MSCs are thought to undergo spontaneous osteogenic differentiation when introduced at the site of damaged bone immediately after harvest (16). This feature allows autologous BMA to be harvested and employed at the time of surgery, circumventing the need for ex vivo cell culture, concentration, and processing (17). In addition to its osteogenic potential, BMA may also possess osteoinductive capacity due to cytokines and growth factors released by the population of cells in the graft (8).
BMA lacks structural integrity and will diffuse away from the implant site if used alone. In order to prevent this, BMA is often mixed with a carrier such as autograft, allograft, ceramics, or demineralized bone matrix (DBM) prior to implantation. When used as graft extender with local bone graft and rhBMP-2, autologous BMA has been shown to elicit a fusion rate of 93% following MIS-TLIF (18). When utilized in MI retroperitoneal approaches, autologous BMA in conjunction with autograft, allograft, DBM, or β-tricalcium phosphate (β-TCP) achieves fusion rates of 93% to 100% (18-21).
Evidence suggests that aspiration technique and volume obtained greatly affect the availability of progenitor cells in the graft material. Muschler and colleagues recommend removal of a maximum of two milliliters of bone marrow from a single site, as greater volumes are overly diluted by peripheral blood (22). BMA may be obtained via needle aspiration of the iliac crest or vertebral pedicles. The concentration of progenitor cells in vertebral aspirates has been shown to be 71% greater than that of samples obtained from the iliac crest (23). In the same study, an age-related decline in progenitor cells was observed in iliac crest-derived BMA. Vertebral aspirates did not demonstrate this trend, and no differences were observed between samples drawn from different vertebral levels (23).
Allogeneic bone graft
Allogeneic bone may be sourced from human cadavers or live donors. Mineralized allograft serves as an osteoconductive scaffold for new bone formation, and may be weakly osteoinductive depending on the preservation of growth factors during processing (17). Its osteogenic potential, however, is removed during processing. Freezing, freeze-drying, or gamma irradiation are required to eliminate its antigenicity, prevent disease transmission, and preserve the graft for later use (24). As an alternative to autograft, allograft preparations avoid donor site morbidity and are available in large quantities at a relatively low cost (24).
While the risk of disease transmission from allograft is extremely rare, instances of pathogen transfer have been reported, including human immunodeficiency virus (HIV), hepatitis B, Clostridium difficile, and other bacterial infections (17). Donor screening and tissue banking procedures have improved since these cases, and the risk of HIV transmission via allograft bone is an estimated 1 in 1.6 million (7). In a review of studies using allograft in MI spine surgery, there were no reported cases of disease transmission (9).
Allograft is chiefly used in combination with autologous bone, BMA, DBM, or growth factor as a bone graft extender in MI procedures (9). In a multicenter review of allograft use in MI transpsoas lateral interbody fusion (MIS-LIF), Ahmadian et al. determined that allograft alone achieves fusion in approximately 87.5% of cases, though materials were not standardized across all institutions (25).
DBM
DBM is an allograft-derived bone graft extender generated through acid extraction of the mineral components of human cadaveric bone (26). This technique preserves material consisting of collagen (primarily type I), noncollagenous proteins, and certain growth factors, including bone morphogenic proteins, transforming growth factor-β, and fibroblast growth factor (8). The amount of viable growth factor remaining after processing differs substantially between the various commercially available DBM products, allowing for considerable variability in osteoinductivity. While DBM is available in several formulations, including powder, gels, and pastes, it lacks mechanical strength and is generally mixed with other bone graft materials to improve osteoconductive capacity and handling properties (27).
Grafts containing autologous iliac crest and DBM have been shown to elicit the same rate of fusion as grafts comprised of ICBG alone in open posterolateral lumbar or lumbosacral fusion (28). Through replacement of autologous bone for DBM, a reduced amount of autologous graft is required, which may mitigate the complications associated with the donor site (28). Further research is required to evaluate the efficacy of similar materials in MI procedures. Across five studies investigating the efficacy of DBM as a bone graft extender in MI posterior interbody fusion, an average fusion rate of 87% was observed. In studies highlighting anterior or lateral approaches, the fusion rate was reported at 97–98% (9).
Recent advances in materials engineering has the potential to further improve DBM as a bone graft extender in spine fusion. Rodriguez et al. describe a model in which defect-matched DBM implants can be custom designed for use in spine surgery. Scaffolds generated with 3D printing technology have been shown to elicit fusion in a rat posterolateral spine model (29). This technology holds promise for use in MI spine surgery as custom construction of malleable implants allows for a precise fit, potentially decreasing time spent preparing graft materials.
Ceramics
Bioceramic scaffolds are synthetic calcium-based products formed by melting inorganic raw materials to generate a crystalline solid (30). Similar to DBM, ceramics are most often employed as bone graft extenders in combination with autologous bone or BMA. Ceramics are also biodegradable, generally non-toxic and non-inflammatory, can be produced on a large scale, and are easily sterilizable (31,32). These materials are brittle and characterized by low tensile strength; however, their porous structure provides a favorable osteoconductive scaffold for new bone growth (8). Macropores permit stem cells migration, adhesion, and differentiation into bone-forming osteoblasts within the scaffold (33).
Commonly employed ceramics include β-TCP, hydroxyapatite (HA), calcium phosphate, and calcium sulfate, which differ in macropore size. In comparison to HA, β-TCP has a greater porosity and individual pore size, which generates a larger surface area and weaker compressive strength. This structure encourages a brisker biologic response, including faster osteoid deposition and bone remodeling. Conversely, HA is dense and is more slowly resorbed after implantation (on the order of years), but provides greater immediate mechanical strength (8).
When used as a bone graft extender with autologous bone in MIS-TLIF, HA achieves rates of fusion comparable to those observed in traditional open cases (86%) (34). In a study of 25 patients undergoing MIS-LIF, graft material comprised of β-TCP, HA, and recombinant human bone morphogenetic protein-2 (rhBMP-2) achieved a 100% fusion rate (35). The osteoinductive effect of rhBMP-2, however, cannot be excluded as a primary contributor to successful fusion. In a randomized controlled trial of single level extreme lateral interbody fusion (XLIF) for degenerative disc disease, patients implanted with rhBMP-2 achieved radiographic fusion more quickly than those who received silicate-substituted calcium phosphate (Si-CaP) grafts (36). After 36 months, all patients in each group achieved fusion, however this lengthy postoperative period may be unfavorable when faster healing alternatives are available. Ceramics used alone in XLIF procedures have been shown to produce a spectrum of fusion rates ranging from 76.3% at mean follow up time of 14.2 months to 93% at 12 months (37).
Bone morphogenetic protein-2 (BMP-2)
BMPs are soluble cytokines in the transforming growth factor-β family. These growth factors act through type I and type II serine-threonine kinase receptors, and exert their downstream effects via the SMAD protein cascade (38). BMPs are highly osteoinductive and promote the differentiation, maturation, and proliferation of osteogenic progenitor cells (39).
The use of rhBMP-2 in MI spine fusion is diverse and includes placement in interbody cages or disc space delivered on collagen sponge, or in combination with other materials (9). When delivered on absorbable collagen sponge (Infuse™), rhBMP-2 has been shown to produce high rates of fusion, including 95% in MIS-TLIF and 100% in XLIF procedures (36,40). Local autograft is commonly supplemented with rhBMP-2 in MIS-TLIF procedures, and this combination has been shown to produce favorable outcomes. In a prospective study of 252 patients, Siddiqui et al. obtained fusion rates of 88.4% and 98.5% at six-month and two-year follow up, respectively. Compared to patients treated with other materials (autograft and/or DBM), the rhBMP-2 group achieved a significantly greater rate of fusion at the six-month interval (88.4% vs. 76.8%, P=0.016), though there was no difference between groups two years (41). In this study, patients underwent fusion with an interbody cage packed with autograft and rhBMP-2, and the remaining disk space was filled with autograft alone (41). rhBMP-2 may also be mixed with the bone graft intended for the disc space as well as the interbody cage. This strategy has been shown in one study to elicit 100% fusion at two-year follow up (42). In addition to the range of available carriers, there is considerable variability in the preferred dose of growth factor, with reports ranging from 1.4 to 12 mg (18,43). Since its FDA approval in 2002, the use of rhBMP-2 has been associated with several side effects, including hematoma formation, heterotopic ossification, retrograde ejaculation, edema, radiculitis, and osteolysis; however, many of these findings were related to surgical technique and naïveté regarding the robust nature of the protein. Given these findings, patients (excluding those with cervical pathologies) should be counseled about the relative risks of growth factor utilization, and individual clinical presentations should determine their use.
Novel therapeutics and techniques
Continued advancement in MI spine surgery will stem from the use of materials specifically engineered to circumvent the problems associated with presently available treatments. Currently, absorbable collagen sponge is the only FDA-approved carrier for rhBMP-2, and while this delivery system is known to achieve high rates of fusion in humans, its use is associated with numerous side effects such as ectopic bone formation, radiculopathy, retrograde ejaculation, and graft subsidence (44). In order to minimize these clinical complications, many research groups have sought to develop a vehicle material with better growth factor localization capacity and improved release kinetics. For example, in 2015, Lee et al. characterized peptide amphiphile (PA) molecules containing a BMP-2 binding motif which readily undergo self-assembly, forming a gel under aqueous conditions (Figure 1) (44). This self-aggregation generates a meshwork of nanofibers akin to the extracellular matrix, and provides a favorable framework for osteogenic cell activity. In vitro assessment of BMP-2 activity within the PA-gel demonstrates enhanced expression of markers of osteoblastic differentiation relative to BMP-2 alone (44). The PA gels are better able to retain BMP-2 compared to collagen sponge, which is known to deliver growth factor in a burst release fashion. Additionally, the ability of PA gels to capture growth factor from surrounding aqueous medium exceeds that of collagen sponge (44). Lee et al. also demonstrated the ability of PA-gel scaffolds to achieve 100% fusion in a rat model using a 10-fold lower dose of growth factor relative to collagen sponge (Figure 2) (44). The dramatic decrease in the requirement for adjunctive rhBMP-2 has the potential to eliminate the negative consequences of growth factor use.
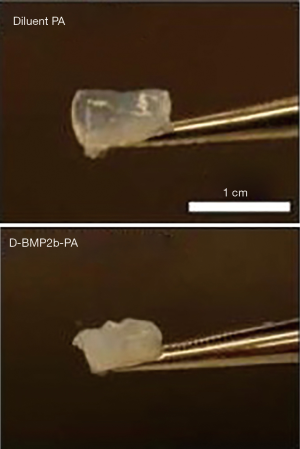
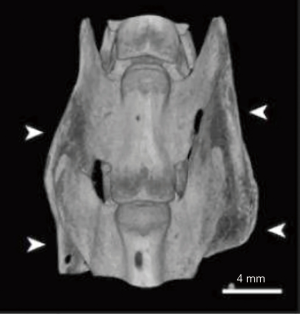
Improvements in the delivery of biologics in the MIS setting have also been investigated. Kleiner et al. outline the shortcomings of conventional bone graft delivery tools used in MI discectomy and fusion, including poor visualization and uneven distribution of graft material once dispensed. They introduced a novel delivery tool designed to overcome these limitations. The delivery cannula has a wedged tip for easier entrance to the disc space, a greater cross-sectional area for improved flow of graft, and two exit portals for lateral, even distribution (45). Coupling enhanced biologic materials such as injectable grafts with improved surgical technique may decrease operative time and improve clinical outcomes.
Discussion
MI lumbar fusion has the potential to reduce patient morbidity, decrease operative time, and reduce the cost of care. The ideal biologic implant must be easily malleable, osteoconductive, osteoinductive, and resorbable. Ideally, grafts will be radiolucent and traceable to allow for radiographic assessment of fusion and correlation with clinical progress (17). Given this demanding rubric, few commercially available grafts exist squarely within these requirements. As MI procedures continue to gain popularity, spine surgeons should endeavor to understand the inherent advantages and disadvantages of the available biologic materials. With the arrival of novel therapeutics, surgeons should be prepared to adopt the use of grafts with demonstrated safety and efficacy that intentionally facilitate the goals of MI surgery.
Acknowledgments
None.
Footnote
Conflicts of Interest: WK Hsu has received research grant from Wright Medical and IP royalties from Stryker; advisory board for the Journal of Bone and Joint Surgery; consulted for Stryker, Medtronic, Mirus, Allosource, Biovenus, Micromedicine, and Agnovos. AC Greene has no conflicts of interest to declare.
References
- Khan NR, Clark AJ, Lee SL, et al. Surgical Outcomes for Minimally Invasive vs Open Transforaminal Lumbar Interbody Fusion: An Updated Systematic Review and Meta-analysis. Neurosurgery 2015;77:847-74; discussion 874. [Crossref] [PubMed]
- Ge DH, Stekas ND, Varlotta CG, et al. Comparative Analysis of Two Transforaminal Lumbar Interbody Fusion Techniques: Open TLIF Versus Wiltse MIS TLIF. Spine (Phila Pa 1976) 2019;44:E555-60. [Crossref] [PubMed]
- Vora D, Kinnard M, Falk D, et al. A comparison of narcotic usage and length of post-operative hospital stay in open versus minimally invasive lumbar interbody fusion with percutaneous pedicle screws. J Spine Surg 2018;4:516-21. [Crossref] [PubMed]
- Huang TJ, Kim KT, Nakamura H, et al. The State of the Art in Minimally Invasive Spine Surgery. Biomed Res Int 2017;2017:6194016. [PubMed]
- Regev GJ, Kim CW, Salame K, et al. A Comparison of Different Minimally Invasive and Open Posterior Spinal Procedures Using Volumetric Measurements of the Surgical Exposures. Clin Spine Surg 2017;30:425-8. [Crossref] [PubMed]
- Roberts TT, Rosenbaum AJ. Bone grafts, bone substitutes and orthobiologics: the bridge between basic science and clinical advancements in fracture healing. Organogenesis 2012;8:114-24. [Crossref] [PubMed]
- Khan SN, Cammisa FP Jr, Sandhu HS, et al. The biology of bone grafting. J Am Acad Orthop Surg 2005;13:77-86. [Crossref] [PubMed]
- Finkemeier CG. Bone-grafting and bone-graft substitutes. J Bone Joint Surg Am 2002;84:454-64. [Crossref] [PubMed]
- Chang KY, Hsu WK. Spinal Biologics in Minimally Invasive Lumbar Surgery. Minim Invasive Surg 2018;2018:5230350. [Crossref] [PubMed]
- Lementowski PW, Lucas P, Taddonio RF. Acute and chronic complications of intracortical iliac crest bone grafting versus the traditional corticocancellous technique for spinal fusion surgery. Orthopedics 2010. [Crossref] [PubMed]
- Lopez GD, Hijji FY, Narain AS, et al. Iliac Crest Bone Graft: A Minimally Invasive Harvesting Technique. Clin Spine Surg 2017;30:439-41. [Crossref] [PubMed]
- Haws BE, Khechen B, Narain AS, et al. Iliac Crest Bone Graft for Minimally Invasive Transforaminal Lumbar Interbody Fusion: A Prospective Analysis of Inpatient Pain, Narcotics Consumption, and Costs. Spine (Phila Pa 1976) 2018;43:1307-12. [PubMed]
- Grabowski G, Cornett CA. Bone graft and bone graft substitutes in spine surgery: current concepts and controversies. J Am Acad Orthop Surg 2013;21:51-60. [Crossref] [PubMed]
- Luis-Ugbo J, Boden SD. Biology of Spinal Fusion. In: Bono CM, Garfin SR. editors. Spine: Orthopaedic Surgery Essentials. Philadelphia: Lippincott Williams & Wilkins, 2004:297-305.
- Defino HL, da Silva Herrero CF, Crippa GE, et al. In vitro proliferation and osteoblastic phenotype expression of cells derived from human vertebral lamina and iliac crest. Spine (Phila Pa 1976) 2009;34:1549-53. [Crossref] [PubMed]
- Barba M, Cicione C, Bernardini C, et al. Adipose-derived mesenchymal cells for bone regereneration: state of the art. Biomed Res Int 2013;2013:416391. [Crossref] [PubMed]
- Campana V, Milano G, Pagano E, et al. Bone substitutes in orthopaedic surgery: from basic science to clinical practice. J Mater Sci Mater Med 2014;25:2445-61. [Crossref] [PubMed]
- Nandyala SV, Fineberg SJ, Pelton M, et al. Minimally invasive transforaminal lumbar interbody fusion: one surgeon's learning curve. Spine J 2014;14:1460-5. [Crossref] [PubMed]
- Hawasli AH, Khalifeh JM, Chatrath A, et al. Minimally invasive transforaminal lumbar interbody fusion with expandable versus static interbody devices: radiographic assessment of sagittal segmental and pelvic parameters. Neurosurg Focus 2017;43:E10. [Crossref] [PubMed]
- Ajiboye RM, Hamamoto JT, Eckardt MA, et al. Clinical and radiographic outcomes of concentrated bone marrow aspirate with allograft and demineralized bone matrix for posterolateral and interbody lumbar fusion in elderly patients. Eur Spine J 2015;24:2567-72. [Crossref] [PubMed]
- Woods KR, Billys JB, Hynes RA. Technical description of oblique lateral interbody fusion at L1-L5 (OLIF25) and at L5-S1 (OLIF51) and evaluation of complication and fusion rates. Spine J 2017;17:545-53. [Crossref] [PubMed]
- Muschler GF, Boehm C, Easley K. Aspiration to obtain osteoblast progenitor cells from human bone marrow: the influence of aspiration volume. J Bone Joint Surg Am 1997;79:1699-709. [Crossref] [PubMed]
- McLain RF, Fleming JE, Boehm CA, et al. Aspiration of osteoprogenitor cells for augmenting spinal fusion: comparison of progenitor cell concentrations from the vertebral body and iliac crest. J Bone Joint Surg Am 2005;87:2655-61. [PubMed]
- Kannan A, Dodwad SN, Hsu WK. Biologics in spine arthrodesis. J Spinal Disord Tech 2015;28:163-70. [Crossref] [PubMed]
- Ahmadian A, Bach K, Bolinger B, et al. Stand-alone minimally invasive lateral lumbar interbody fusion: multicenter clinical outcomes. J Clin Neurosci 2015;22:740-6. [Crossref] [PubMed]
- Lee KJ, Roper JG, Wang JC. Demineralized bone matrix and spinal arthrodesis. Spine J 2005;5:217S-223S. [Crossref] [PubMed]
- Tilkeridis K, Touzopoulos P, Ververidis A, et al. Use of demineralized bone matrix in spinal fusion. World J Orthop 2014;5:30-7. [Crossref] [PubMed]
- Cammisa FP Jr, Lowery G, Garfin SR, et al. Two-year fusion rate equivalency between Grafton DBM gel and autograft in posterolateral spine fusion: a prospective controlled trial employing a side-by-side comparison in the same patient. Spine (Phila Pa 1976) 2004;29:660-6. [Crossref] [PubMed]
- Rodriguez RU, Kemper N, Breathwaite E, et al. Demineralized bone matrix fibers formable as general and custom 3D printed mold-based implants for promoting bone regeneration. Biofabrication 2016;8:035007. [Crossref] [PubMed]
- Jarcho M. Calcium phosphate ceramics as hard tissue prosthetics. Clin Orthop Relat Res 1981.259-78. [PubMed]
- Kadam A, Millhouse PW, Kepler CK, et al. Bone substitutes and expanders in Spine Surgery: A review of their fusion efficacies. Int J Spine Surg 2016;10:33. [Crossref] [PubMed]
- Nickoli MS, Hsu WK. Ceramic-based bone grafts as a bone grafts extender for lumbar spine arthrodesis: a systematic review. Global Spine J 2014;4:211-6. [Crossref] [PubMed]
- Khan SN, Fraser JF, Sandhu HS, et al. Use of osteopromotive growth factors, demineralized bone matrix, and ceramics to enhance spinal fusion. J Am Acad Orthop Surg 2005;13:129-37. [Crossref] [PubMed]
- Yoo JS, Min SH, Yoon SH. Fusion rate according to mixture ratio and volumes of bone graft in minimally invasive transforaminal lumbar interbody fusion: minimum 2-year follow-up. Eur J Orthop Surg Traumatol 2015;25 Suppl 1:S183-9. [Crossref] [PubMed]
- Dakwar E, Cardona RF, Smith DA, et al. Early outcomes and safety of the minimally invasive, lateral retroperitoneal transpsoas approach for adult degenerative scoliosis. Neurosurg Focus 2010;28:E8. [Crossref] [PubMed]
- Pimenta L, Marchi L, Oliveira L, et al. A prospective, randomized, controlled trial comparing radiographic and clinical outcomes between stand-alone lateral interbody lumbar fusion with either silicate calcium phosphate or rh-BMP2. J Neurol Surg A Cent Eur Neurosurg 2013;74:343-50. [Crossref] [PubMed]
- Alimi M, Navarro-Ramirez R, Parikh K, et al. Radiographic and Clinical Outcome of Silicate-substituted Calcium Phosphate (Si-CaP) Ceramic Bone Graft in Spinal Fusion Procedures. Clin Spine Surg 2017;30:E845-52. [Crossref] [PubMed]
- Katagiri T, Watabe T. Bone Morphogenetic Proteins. Cold Spring Harb Perspect Biol 2016. [Crossref] [PubMed]
- Chen G, Deng C, Li YP. TGF-β and BMP signaling in osteoblast differentiation and bone formation. Int J Biol Sci 2012;8:272-88. [Crossref] [PubMed]
- Dahdaleh NS, Nixon AT, Lawton CD, et al. Outcome following unilateral versus bilateral instrumentation in patients undergoing minimally invasive transforaminal lumbar interbody fusion: a single-center randomized prospective study. Neurosurg Focus 2013;35:E13. [Crossref] [PubMed]
- Siddiqui MM, Sta Ana AR, Yeo W, et al. Bone Morphogenic Protein Is a Viable Adjunct for Fusion in Minimally Invasive Transforaminal Lumbar Interbody Fusion. Asian Spine J 2016;10:1091-9. [Crossref] [PubMed]
- Park P, Foley KT. Minimally invasive transforaminal lumbar interbody fusion with reduction of spondylolisthesis: technique and outcomes after a minimum of 2 years' follow-up. Neurosurg Focus 2008;25:E16. [Crossref] [PubMed]
- Tsahtsarlis A, Wood M. Minimally invasive transforaminal lumber interbody fusion and degenerative lumbar spine disease. Eur Spine J 2012;21:2300-5. [Crossref] [PubMed]
- Lee SS, Hsu EL, Mendoza M, et al. Gel scaffolds of BMP-2-binding peptide amphiphile nanofibers for spinal arthrodesis. Adv Healthc Mater 2015;4:131-41. [Crossref] [PubMed]
- Kleiner JB, Kleiner HM, Grimberg EJ Jr, et al. Evaluation of a novel tool for bone graft delivery in minimally invasive transforaminal lumbar interbody fusion. Med Devices (Auckl) 2016;9:105-14. [Crossref] [PubMed]